The lateral geniculate nucleus: the gateway to conscious visual perception
Conscious perception requires the visual information that passes through the dorsal lateral geniculate nucleus (LGN) in primates. Although the retina sends axons to many subcortical nuclei, only the pathway from the retina to LGN to cortex is critical to visual awareness.
The LGN is a distinctively layered structure and is located at the posterior lateral margin of the dorsal thalamus ( Fig. 29.1 ). Although there is agreement that the LGN provides the key visual gateway to cortex, there is less agreement over LGN function. , The main reason is that receptive field properties of LGN cells are so similar to those of their retinal ganglion cell inputs. Given that visual signals are transformed in other visual areas it is puzzling that a similar transformation can not be identified in the LGN. The most reasonable explanation is that the main role of the LGN is to regulate the flow and strength of visual signals sent to V1. Evidence indicates that this regulation is much more complex than simply opening and closing a single gate. The LGN and visual cortex, working together, form part of a dynamic system designed to rapidly and efficiently process the most useful visual signals for survival. The parallel processing of visual information, elaborate circuitry and many extraretinal inputs, including the massive visual cortical feedback to the LGN, all suggest that visual signals are regulated in a variety of specific and general ways within the LGN.

The purpose of this chapter is to illuminate the structure and function of the LGN by first reviewing LGN anatomy and physiology and then current controversies over signal processing. The field has moved from static descriptions of LGN cell receptive field properties to dynamic descriptions that take into account eye movements, motor planning, arousal level, attention and possibly even more complex information concerning visual memories. In the following two sections, we describe the basic architecture, connections and neurochemistry of the LGN. The next four sections consider the LGN in a functional context beginning with the basics of signal processing in section four. Then we consider the potential functional impact of attention, motor planning, and binocular rivalry. The final section summarizes key points.
Overview of lateral geniculate anatomy
Layers and maps
The LGN is an elongate layered structure with each layer representing the opposite visual hemifield ( Fig. 29.2 ). Within each layer the opposite hemifield is represented such that the superior and inferior visual fields are located toward the lateral and medial zones of the layer, respectively, and the central (toward the fovea) and peripheral visual fields are located, respectively, at the posterior and anterior zones of each layer. Each layer receives monocular input from the retina and only those layers receiving input from the contralateral eye (nasal retina) represent the entire contralateral visual hemifield since LGN layers receiving input from the ipsilateral eye (temporal retina) cannot represent the monocular portion of the hemifield. This means that the ipsilateral layers are always shorter in all dimensions than the corresponding contralateral layers ( Fig. 29.2 ). The maps in each layer are retinotopically aligned such that each point in visual space is represented along a line perpendicular to the layers. The alignment of the maps is so precise that a cell-free gap, representing the optic disk (see arrows in Figs 29.1B and 29.2 ), exists in all contralaterally innervated layers in order to maintain retinotopic alignment with the ipsilaterally innervated layers. This is because the optic disk contains no receptors and is located in the nasal retina.

There are three types of cell layers, those with large cells (the magnocellular or M layers), those with medium size cells (the parvocellular or P layers) and those with very small cells (the koniocellular or K layers). All primates have at least two P and two M layers with K layers lying below each M and P layer. In humans and some other primates, each P layer can split into two or more layers in the portion of the LGN that represents central vision (∼2–17 degrees). The normal human LGN can contain as few as two or as many as six P layers.
Among primates, the laminar organization and topography of the LGN has been studied in the most detail in the macaque monkey. Malpeli and colleagues , performed careful reconstructions of the entire LGN documenting the retinotopic organization and number of M and P cells in each LGN layer (K cells were not counted). These studies have shown that in macaque monkeys the representation of the central visual field is magnified in the LGN ( Fig. 29.2 and see Fig. 29.5 below). The magnified representation of the central few degrees in the LGN is easy to understand given that each LGN cell receives input from approximately one to three retinal ganglion cells and ganglion cell density is much higher in central retina. Only within the portion of the nucleus representing eccentricities from 2 to 17 degrees do the two P layers split to represent four or more P layers, an arrangement which may reflect an increase in the diversity of retinal ganglion cells representing this portion of visual space. Classical textbook sections of the LGN typically are taken from this region of the LGN which shows four P layers and two M layers; as mentioned, we now know that K layers lie below each P and M layer as depicted for the macaque monkey in Figure 29.3 .

Cell classes
All LGN cells can be grouped into two principal cell classes, relay cells that send an axon to visual cortex and interneurons whose axons remain within the LGN. LGN relay cells use the transmitter glutamic acid (glutamate) and interneurons use the transmitter gamma-amino butyric acid (GABA). Relay cells and interneurons occur in a ratio of approximately 4:1 throughout the LGN and exhibit distinct dendritic morphologies. The LGN contains several classes of interneurons whose general role is to regulate signals that will ultimately pass to cortex from the retina via relay cells. The M, P, and K cells mentioned previously are all classes of relay cells and, in addition to size, can be distinguished based on dendritic morphology, calcium binding protein content, physiological properties, and axonal projection patterns to cortex. P and M LGN cells play a major role in setting up the properties of V1 cells, but it remains controversial as to whether P and M cells belong to just two classes. Evidence from retinal inputs, axon projection patterns and physiology indicate that there are subclasses of P and M cells that perform somewhat different functions in V1 (see also below). It is even more likely that K cells are not a single LGN cell class given the diversity of their connections to cortex (see also below).
Inputs: the retina
Classically it was argued that there were two pathways from the retina to the LGN, the P and the M pathways; all other retinal ganglion cells were presumed to project to other subcortical nuclei. Evidence from more recent studies has shown that 10 or more different types of retinal ganglion cells send axons to the LGN based primarily on morphology ( Fig. 29.4 ). This finding complicates the picture because it becomes unclear whether K, M, and P LGN cells, as described above, receive unique inputs from only one class of ganglion cell or more than one class. It is evident from projection patterns of LGN axons in V1 that at least 10 axon classes have been identified that could support the argument that each retinal ganglion cell class has a unique conduit to cortex via a labeled line through the LGN (see also Fig. 29.6 below).

Inputs: extraretinal sources and cortical feedback
In addition to retinal input, LGN cells receive input from a variety of both visual and non-visual extraretinal sources. These extraretinal afferent sources provide a much larger input to the LGN, in terms of synapse number, than does the retina (see review ). These extraretinal inputs also regulate the flow of information in the LGN in a variety of ways given the different transmitters they contain and the number of transmitter receptors that exist on LGN neurons ( Fig. 29.5 ). Input from extraretinal visual sources maintains retinotopic fidelity, meaning, regions representing a common point in visual space are connected. Extraretinal visual input to the LGN has been documented from the following areas in primates with their transmitter type in parentheses: primary visual cortex (glutamate), some extrastriate areas (likely glutamate), superior colliculus (glutamate), pretectum (GABA), parabigeminal nucleus (acetylcholine or ACh), and the visual sector of the thalamic reticular nucleus (GABA). Of these extraretinal visual sources the two major sources, in terms of synapse number, come from the thalamic reticular nucleus and the primary visual cortex. The input from the thalamic reticular nucleus has been studied in great detail, and together with the primary visual cortex, provide retinotopically localized regulation of LGN signals to cortex. The massive cortical feedback pathway is highly specific in that cells in different subdivisions of layer VI of V1 send axons to either the P or M LGN layers but not both. This feedback also targets the K LGN layers and the thalamic reticular nucleus but only via axon collaterals destined for either the P or M LGN layers at least in owl monkeys.

Extraretinal input to the LGN also arrives from several non-visual sources. The most well studied of these afferents are the cholinergic afferents from the pons that innervate all LGN layers and also contain the transmitter nitric oxide at least in the cat. Other afferent sources include a serotonergic input from the dorsal raphé nucleus and a histaminergic input from the hypothalamus.
Outputs: projections to V1 and beyond
In primates the bulk of the efferent axonal output from the LGN terminates within the primary visual cortex and the visual sector of the thalamic reticular nucleus (TRN). Although not documented in primates, in cats the projection to the equivalent of the visual sector of the TRN comes from collateral branches of LGN relay cell axons not from a separate projection that goes solely to this thalamic nucleus. A smaller efferent projection from the LGN also has been reported to terminate in several extrastriate visual areas. The most well documented of these projections is to the middle temporal area (MT). This projection appears to originate from K LGN cells, based upon double labeling studies using retrograde tracers and immunocytochemistry for calbindin, and arises from K cells that do not send collateral projections to V1. Although this projection involves only about 1 percent of LGN cells, it has been implicated in the residual vision referred to as “blindsight” in patients that have lost their primary visual cortex ( Box 29.1 ).
Cats (A; courtesy of Julie Mavity-Hudson) show only a slight loss in visual acuity following bilateral removal of primary visual cortex and tree shrews (B; courtesy of Robert Strawn) (the closest living relatives of primates) show no deficit in acuity following similar lesions. In both of these species it has been argued that vision is preserved via alternative subcortical routes to extrastriate cortex. Humans, however, appear to be completely blind following similar damage to V1. Nevertheless, it has been argued by some investigators that residual vision within the “blind” field (so called “ blindsight ”) exists even in patients with damage or complete loss of V1. Controversy persists, however, over whether such vision simply reflects small remaining pieces of V1 that are functional or is really the result of a separate functional pathway to extrastriate cortex as has been demonstrated in cats and tree shrews. Even among those who believe that a functional retinal route exists in humans and other primates that bypasses V1 there is debate as to whether this route passes through the LGN via direct projections from koniocellular (K) cells to extrastriate cortex or involves a pathway from the retina to the superior colliculus to the pulvinar to extrastriate cortex as has been demonstrated in tree shrews.

In primates, studies have shown that P cells send axons principally to the middle and lower tier of layer IVC of V1 (using the nomenclature of Brodmann ). In addition, some P cells in macaque monkeys may send axons to IVA. The projection to layer IVA appears to be a specialization of only certain primates since it is absent in chimpanzees and likely also humans. M cells send their efferent axons to the middle and upper tier of layer IVC of primary visual cortex. Finally, K cells send their axons to cortical layers IVA (in macaque monkeys) and IIIB/IIIA where they terminate in patches of cells whose mitochondria contain high amounts of cytochrome oxidase (CO), the “CO blobs” and to layer I (see review ). Some M and P cells also have sparse projections to layer VI in V1. The pathways to V1 are summarized in Figure 29.6 .

LGN circuits: How are visual signals regulated?
Feedback and feedforward pathways
Visual signals within the LGN are regulated by circuits involving two groups of inhibitory neurons, local inhibitory neurons and TRN inhibitory neurons. These inhibitory neurons are connected in feedforward and feedback circuits to LGN relay cells involving, respectively, retinal input to local inhibitory cells and relay cell input to TRN neurons as shown schematically in Figure 29.7A . The feedforward pathway involves a unique synaptic arrangement referred to as a triad in which a retinal terminal synapses upon a relay cell dendrite and the dendrite of an inhibitory interneuron ( Fig. 29.7B ). The dendrite of the inhibitory interneuron, in turn, makes a dendro-dendritic synapse on the same relay cell dendrite. In contrast, in feedback inhibition the relay cell synapses on the dendrite of a TRN neuron that then sends an inhibitory connection back to terminate on the dendrite of the same relay cell ( Fig. 29.7A ). Given that feedforward and feedback circuits can influence how retinal input is modulated, further documentation of ultrastructural differences in the circuits within the different layers of the primate LGN will become critical to the development of accurate physiological models.

Circuit neurochemistry
Although the feedback and feedforward circuits to LGN relay cells form the basic architecture for controlling visual signals, the final information that is sent on to the visual cortex is dependent in complex ways on all of the inputs that can impinge upon this circuitry and the variety of transmitter receptors that are activated by these inputs. Given space limitations we can only give a few examples here. Many of the same inputs that contact LGN relay cells are known to also contact inhibitory interneurons within the LGN or within the TRN although the transmitter receptors in some cases differ. The visual cortex is known to provide the most massive extraretinal input to the LGN. This glutamatergic input arriving from cells in layer VI of cortex activates both ionotropic and a specific class (group 1) of metabotropic glutamate receptors, both of which can directly depolarize relay cells although (importantly) with very different time courses and potentially different effects on the firing pattern of LGN relay cells (see also ). Since cortical axons also synapse with inhibitory interneurons and TRN neurons that themselves have both types of receptors, the net output from the relay cell can not easily be predicted even from a simple circuit involving feedback from visual cortex.
A second major input to the LGN comes from cholinergic neurons in the pons (the parabrachial region in the cat). These cholinergic fibers innervating the interneurons and relay cells of the LGN and the TRN also are capable of releasing a second transmitter, nitric oxide. The impact of this input on the output of visual signals to cortex is complicated by the fact that ACh can activate both fast ionotropic nicotinic receptors and slower metabotropic M1 receptors on relay cells; both result in relay cell depolarization. These same ACh-containing pontine axons activate M2 receptors on interneurons and TRN cells resulting in hyperpolarization of these cells. The net impact of this ACh input on LGN relay cells, therefore, is generally excitatory since relay cells are directly depolarized and feedback and feedforward inhibition blocked. Under this condition one would predict an increased transfer ratio of retinal signals to cortex. In contrast, activation of the inhibitory circuitry would lower the transfer ratio and, under some circumstances, could change the temporal structure of signals arriving from the retina. The cortical and pontine inputs and their receptors in the LGN just described are only two examples that have been worked out for the cat LGN. The number of axonal inputs to the LGN and the variety of effects that each can have depending upon which receptor is activated argues strongly that LGN gating is not equivalent to simply opening or closing a connection between the retina to the cortex.
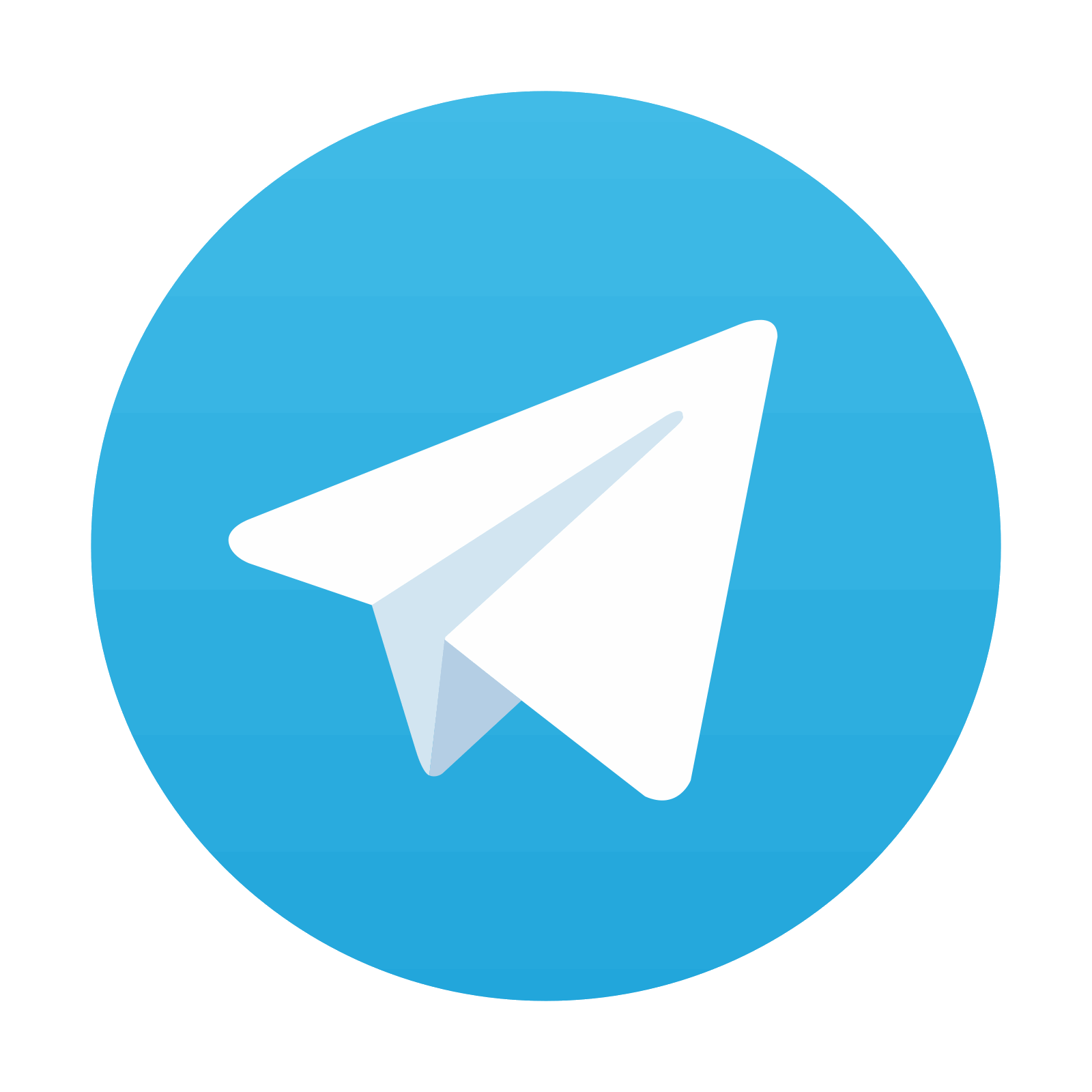
Stay updated, free articles. Join our Telegram channel
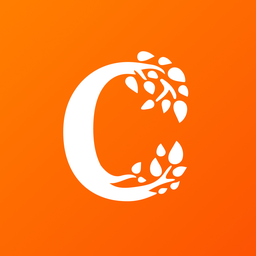
Full access? Get Clinical Tree
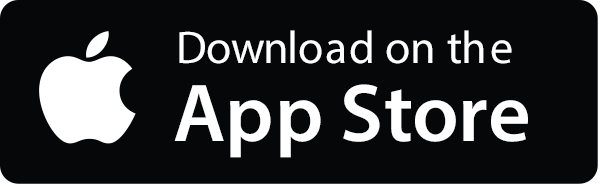
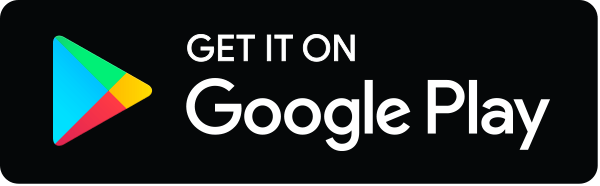