Retinal rods and cones are highly specialized neurons that respond to light with an electrical signal (see Chapter 18 ) and provide the sensory input for vision. In contrast to most other neurons, rods and cones maintain a relatively depolarized membrane potential at rest (in darkness) and when stimulated (by light) decrease Na + entry by closing ion channels. In turn, the resultant hyperpolarization closes Ca 2+ channels at the synapse and the ensuing fall in intracellular Ca 2+ reduces an ongoing vesicular release of neurotransmitter onto second-order neurons (see review ). This chapter describes the signaling properties of rods and cones, which subserve vision in dim and bright light, respectively. An overview of the voltage changes induced by light will be followed by a description of the properties of the photocurrent from which the voltage response originates and then an explanation of how voltage-dependent, inner segment conductances shape the final voltage response. For readers interested in the underlying molecular designs and mechanisms, summaries on ion channels and exchangers are included. Even though the retinal photoreceptors of all vertebrates operate similarly, there are some qualitative as well as quantitative differences. So wherever possible, we will focus on primate photoreceptors.
Photovoltage response to flashes
In darkness, rods and cones maintain a membrane potential near −40 mV. When stimulated by flashes, rods and cones do not “fire” action potentials but instead respond with graded hyperpolarizations ( Fig. 19.1 ) that in some cells, exceed 25 mV. The hyperpolarization then spreads passively to the synapse. In comparing rod and cone responses, the latter are considerably faster and require many more photons. Photoisomerization of 75 rhodopsins gives rise to a half maximal response in rods whereas in cones, it takes close to a thousand photoisomerizations (estimated from , ).

The amplitude of the single photon response in rods is about a millivolt, a few percent of the maximal response. Its time to peak of 200 ms or more is relatively slow considering that Olympic sprinter Usain Bolt covered more than two meters in that span of time. The response recovers even more slowly and may take over a second to disappear. The slow time course of the single-photon response provided compelling evidence for an internal, second messenger(s) and for amplification steps in the signaling pathway. The duration can be specified as an integration time, calculated by dividing the response integral by the peak amplitude. For rods, the integration time of the quantal response is several hundred ms. Although cones are less sensitive than rods, they too “respond” to single photons, but the amplitude of their quantal response is much smaller and does not emerge from the baseline noise. The cone response is faster, with a time to peak of 30 ms and an integration time of only 25 ms. , Response kinetics and sensitivity are intimately linked and the relationship will be discussed below (see also Chapter 18 ). The waveforms of rod and cone flash responses change with flash strength. Responses peak sooner and the initial recovery is faster as the flash strength increases but with responses greater than half maximal, recovery stalls to a plateau before the final recovery. In cones, the recovery overshoots the baseline before the final recovery, while in rods, the plateau slowly returns to baseline without an overshoot.
Background light attenuates the response to a flash as the cells adapt (for discussions of the underlying mechanisms, see Chapters 18 and 20 ). The dim flash response reduces in size by two-fold with a background producing ~150 photoisomerizations per second in rods and ~8700 photoisomerizations per second in cones. The latter value obtains for an isolated cone. In reality, light has a greater effect on the cone response because of electrical coupling between rods and cones in the retina (see Chapter 22 ). Background light quickens flash responses in rods, but has little effect on response kinetics in cones (ignoring the rod component of the response in cones communicated electrotonically).
Photocurrent response to flashes
The photon response derives from a decrease in the number of open cyclic nucleotide gated (CNG) channels that perturbs the flow of ions across the plasma membrane and shifts the transmembrane potential to more negative voltages. CNG channels are located exclusively in the outer segments of rods and cones. In darkness, a small fraction of the channels are in the open state, allowing an influx of cations. The intracellular concentration of Na + is low while that of K + is high relative to the extracellular space due to the action of Na + ,K + -ATPases in the inner segment. Thus Na + and also some Ca 2+ ions flow in through the outer segment channels. K + ions flow out through leak and voltage-gated ion channels in the inner segment to complete the electrical circuit, which is referred to as the “dark” or circulating current ( Fig. 19.2 ). Light absorbed by a visual pigment molecule in the outer segment closes CNG channels in an annulus of plasma membrane surrounding the site of photon absorption on the disk membrane.

When two photons are absorbed, it is unlikely for both absorptions to occur in close proximity. The response to each photon is generated independently and the overall response is twice as big. In other words, dim flashes lie within a linear operating range wherein the response simply scales with the flash strength. However, with increasing numbers of photon absorptions, local effects begin to overlap so fewer channels are closed per photon absorbed. With enough photons, all of the channels are closed and the rod response is maximal ( Fig. 19.3 ). Hence the normalized response grows with flash strength according to a saturating exponential function:
r / r max = 1 − exp ( − k f i )

As is the case with photovoltage, photocurrent responses speed up with flash strength though the effect is less pronounced in cones. , Responses are faster in cones than in rods (note the difference in time scales in Fig. 19.3A and C ) and have a marked undershoot (although cf. refs , ). Responses of different cone types are indistinguishable in waveform. However, the photovoltage and photocurrent responses of rods and cones are not mirror images of each other; photocurrent responses lack the “nose” in the photovoltage response to brighter flashes.
Once the flash is bright enough to close all of the CNG channels, more light cannot produce a larger response, so instead, the duration of the photocurrent response increments with the natural logarithm of the flash strength ( Fig. 19.3D ). The basis for this behavior is that a single molecular process, namely the shutoff of transducin (see also Chapter 18 ), that has a stochastic, exponential time course , dominates the recovery from phototransduction activation in rods. The slope of the saturation function or “Pepperberg Plot” yields the dominant time constant of about ~0.2 s (a similar value has been reported by many others for murine rods). The identity of the rate-limiting step in the recovery of the cone response has not been firmly established but its time constant is an order of magnitude faster (T. Kraft, personal communication).
Detecting single photons
Rods count single photons. In order to do so, they must satisfy several conditions: noise must be minimal and quantal responses must be reproducible and highly amplified. Amplification is achieved by cascading several enzymatic reactions (see Chapter 18 ). Each chemical reaction takes time, hence the decrement in the circulating current after photon absorption is delayed by a few ms. Then the response rises to a peak amplitude of 0.1 to 0.7 pA approximately 200 ms later reflecting the suppression of the circulating current by a few per cent. With the cascade working as fast as it can, the size of the response is determined by when shutoff and recovery processes kick in. In rods, hundreds of CNG channels are closed during the single photon response. Na + ions traverse the channel at a rate of ~10 4 s −1 (see review ), so one photon blocks the entry of a million Na + ions. Responses to brighter flashes in rods and in cones appear with a shorter delay and rise more steeply because many more photoexcited rhodopsins are activating the phototransduction machinery. But if the single photon response in cones is considered, its early rise is actually pretty similar to that in rods. However, the cone response starts to shut off in 50 ms or less at a time when it has only reached an amplitude of a few tens of fA.
It is imperative that single photon responses do not vary in size or duration because if they did, the rod would be unable to distinguish one large, slow quantal response from two or more small, brief quantal responses occurring close together in time. The coefficient of variation of the rod response, defined as the quotient of the standard deviation divided by the mean amplitude, has a low value of 0.2. When stimulated by dim flashes, the rod does not respond the same way every time, but the unpredictability arises from the Poisson distribution of photon absorptions, rather than from single photon response variability. Whenever the rod does respond, the amplitude is quantized ( Fig. 19.4A ).

In darkness, there are two physiological kinds of electrical fluctuations in the current baseline: discrete noise and continuous noise ( Fig. 19.4B ) , Discrete noise arises from thermal isomerizations of rhodopsin. Even though rhodopsin has a half life of ~300 years at body temperature, a rod contains so many copies (around a hundred million) that one spontaneously activates every minute or two. Cone visual pigments are far less stable than rod pigments, making single photon detection impossible. Continuous noise springs from the spontaneous activity of PDE and is much more prevalent than discrete events in rods but typically has a lower amplitude. The continuous noise amplitude distribution is approximately Gaussian so, occasionally, there is a single-photon-response-like event. However, such events are inconsequential because they take place ten times less frequently than the thermal isomerizations of rhodopsin.
Thermal isomerizations of rhodopsin limit the detection of very dim light, because real photons cannot be distinguished from virtual ones. To guard against false alarms, the visual system relies on coincidence detection. A single photon response in one rod does not suffice for vision; a few rods in a small cluster of the retina must each generate a single photon response within a certain period of time before a flash is “seen”. The temporal requirement is specified by the integration time of the response. Thus shortening the integration time detracts from sensitivity of the system. This consideration also applies below, when rods and cones respond to steps of light. The integration time for rods is ~300 ms, while for cones, it is ~20 ms (segment preceding undershoot ).
Thus rods are well suited for single photon detection. Oddly though, rods sometimes generate single photon responses that are truly aberrant. , For reasons unknown, one photoexcited rhodopsin out of several hundred fails to shut off properly , and gives rise to a two-fold larger than average response that can last for a very, very long time ( Fig. 19.5 ). Aberrant response durations are exponentially distributed with a mean value of about 4 s, but some last for tens of seconds. Aberrant responses have little impact on photon counting due to their rarity, yet they prolong the recovery after exposure to bright light (flashes and steps), leaving rods vulnerable to saturation ( Box 19.1 ).

Excessive phototransduction cascade activity in photoreceptors can interfere with vision and lead to retinal disease (see review ). For example, mutations that target rhodopsin kinase (see Chapter 18 ), can cause a form of night blindness known as Oguchi disease. Mutant mouse rods lacking rhodopsin kinase generate aberrant single photon responses like those shown in Fig. 19.5 for every rhodopsin isomerization. Cones are only mildly affected in the disease, because they express a second type of rhodopsin kinase. , Patients with defects in the nuclear receptor NR2E3 develop enhanced S cone syndrome in which there is a higher prevalence of blue cones in the retina at the expense of red and green cones. Very interestingly, the blue cones in these patients fail to express both types of rhodopsin kinases. Hence flash responses of their blue cones (but not red or green cones) do take an abnormally long time to recover.
Mutations in arrestin, the protein normally responsible for quenching photoexcited rhodopsin’s activity (see Chapter 18 ), can also cause Oguchi disease. Flash responses from rods of mutant, arrestin knockout mice are very prolonged ( Fig. 19.8 ). Since longer-lasting responses enhance absolute sensitivity, it might at first seem surprising that the mutations in either rhodopsin kinase or arrestin should lead to night blindness. The problem is that the mutant rods saturate at very low light levels and then take an inordinately long period of time and much dimmer conditions to recover from saturation. In theory, Oguchi patients with rhodopsin kinase or arrestin mutations might actually see better than normal persons under the dimmest conditions when given adequate time for dark adaptation. Photopic vision is largely spared because cones express a unique arrestin. ,

Mutations in either RGS9 or R9AP can sabotage the timely shutoff of transducin (see Chapter 18 ). Because these two proteins are used in rods and cones, there is night blindness and a problem in daytime vision. The ability of cones to light adapt enables them to escape saturation, so photopic vision is still possible. But long lasting photoresponses translate into a disturbing persistence in sensation and the person has difficulty following moving objects and adjusting to luminance changes, a condition termed bradyopsia.
Genetic defects in RPE65, the isomerase that converts all- trans to 11- cis retinal (see Chapter 13 ) can prevent the de novo synthesis of rhodopsin. The persistent presence of catalytically active apo-opsin (rhodopsin lacking 11- cis retinal – essentially equivalent to bleached rhodopsin) gives rise to Leber congenital amaurosis, an especially severe form of retinal degeneration. RPE65 knockout mouse rods exist in an inescapable state of light adaptation, with greatly attenuated circulating current and smaller, faster flash responses ( Fig. 19.9 ).

Photocurrent response to steady light
The rod response to dim, steady light summates individual single photon responses. But with brighter light, adaptation within the outer segment causes the step responses to fall short of the amplitude expected from a simple saturation behavior (see Chapter 20 ). Midrange responses droop as additional adaptational mechanisms with a slower time course reduce the cascade gain and cause the stimulus–response relation to rise even more gently ( Fig. 19.6 ). Light producing 300–600 photoisomerizations per second decreases the circulating current in darkness by 50 percent. , In contrast, the peak of the cone response to steps nearly does obey simple saturation. A hundred ms in the light later, however, cone responses begin to droop as they too adapt. For dim steps, the droop reduces the amplitude to less than half that at the peak. The initial droop is typically larger and faster in cones than in rods.

In steady light, incremental flashes give rise to responses in rods that are smaller ( Fig. 19.7 ) and recover more rapidly than in darkness, another manifestation of light adaptation (see Chapter 20 ). Flash response kinetics change very little with background light in primate cones, differing in this respect from cones of cold-blooded vertebrates. Light producing one to a few hundred photoisomerizations per second reduces flash sensitivity to half its value in darkness in rods, where the value may increase slowly over tens of seconds in the light. , Adaptation is more powerful in cones, for which 10,000 or more photoisomerizations per second reduce sensitivity two-fold. , Mammalian rods adapt over several log units of background light intensity before saturating. Amphibian rods adapt over an even greater range of intensities at least in part because they lack aberrant responses.

Light that is bright enough to bleach a significant fraction of visual pigment causes rods to behave for a period as if they were being exposed to a virtual, “equivalent light” that lingers after the light is removed (see review ). Rods adapt to the equivalent light with a reduction in circulating current and accelerated flash response kinetics as well as a profound loss of flash sensitivity exceeding that expected from the decrease in photon capture. It turns out that bleached rhodopsin constitutively activates the phototransduction cascade. Although the activity of a single bleached rhodopsin is miniscule, the summated activity over hundreds of millions of bleached rhodopsin molecules is substantial. The equivalent light fades as visual pigment regenerates (see Chapter 13 ). Circulating current after full bleach recovers halfway in 15 min and is fully restored in ~25 min. Cones are not impaired in this way by bleaching; , their bleached pigment seems to be inactive (although the situation is not yet clear for bleached blue cone pigment ). That means that when a very bright light is turned on, cones may saturate, but they actually manage to recover circulating current during the exposure as loss of some of their visual pigment by bleaching lowers the ongoing rate of photon capture. Even after extensive bleaching, cones regain their full circulating current and the reduction in sensitivity scales in proportion to the loss in photon capture. Cone pigment regeneration is faster than in rods, with full sensitivity returning after complete bleach within minutes. So while cones lack the absolute sensitivity of rods, they are better equipped to operate over a wide range of brighter light intensities without saturating.
Action spectra of rods and cones
Rods and cones respond to a wide range of wavelengths ( Fig. 19.10 ), their action spectra being determined by the spectral absorptions of the visual pigment they express. For a given number of photons, the response amplitude varies with wavelength because the probability of absorption by the visual pigment varies with wavelength. The response itself to a photoisomerization is independent of wavelength. Rods respond maximally to ~493 nm (blue-green light). , There are three types of cones, commonly referred to as blue, green and red with maxima near 430 nm (violet light), 530 nm (green light) and 560 nm (greenish-orange light), respectively. , Since the names do not correspond to the colors of the maxima in every case, many prefer the designations: short-wavelength sensitive or S, middle-wavelength sensitive or M and long-wavelength sensitive or L ( Box 19.2 ).

Normal color vision requires the proper electrical signaling of light by all three cone types. Evolutionarily, the red and green cone pigment genes arose from a duplication event and they lie together on the X chromosome. Their high sequence similarity has caused “confusion” during crossing over in gametogenesis creating within the population, hybrid genes, fragmented genes and variable numbers of copies of pigment genes on the chromosome ( Fig. 19.11 ). In persons with many genes in the array, expression of the first two predominates. Yet some of these individuals and also women with different sets of pigment gene variants on their X chromosomes actually possess more than three cone types in their retinas. The genetics help to explain the substantial individual variation in color vision in “normal” persons, anomalous color vision as well as some forms of color “blindness” (see reviews , ). The last category includes extreme cases where failure to express one or both types of pigment genes on the X chromosome gives rise to dichromacy or blue cone monochromacy, respectively.

CNG channel and Na + /K + ,Ca 2+ exchanger
Two types of molecules are the principal mediators of ionic movement across the outer segment plasma membrane: the CNG channel and the Na + /K + ,Ca 2+ exchanger. CNG channels are heterotetramers consisting of CNGA1 and CNGB1 subunits ( Fig. 19.12A ) in a ratio of 3:1, respectively in rods and CNGA2 and CNGB3 subunits in a B3-B3-A3-A3 arrangement in cones. ( Box 19.3 ) Although CNG channels are members of the same superfamily as voltage-gated K + channels, they are only mildly voltage-dependent. Instead, CNG channels in rods and cones are directly gated by cGMP. , Cyclic AMP also works, but with a 50-fold lower K 0.5 reflecting the reduced affinity of the channel for cAMP and the reduced efficacy in opening once cAMP has bound. While other ligand-gated ion channels in the body desensitize in the continued presence of their ligand, the CNG channel does not have any intrinsic mechanisms of desensitization and thus steadily monitors the [cGMP] in darkness. ,

Rod monochromacy is an autosomal recessive condition presenting with achromatopsia, poor visual acuity, photophobia and nystagmus. It is fairly rare, affecting fewer than 1 in 30,000 persons, although the prevalence is as high as 1 in 10 amongst the native population of Pingelap (see review for an interesting account). In most cases, the condition is a channelopathy, caused by mutations in CNGA3 or CNGB3 channel subunits that disable phototransduction in cones (see reviews , ). Cases of incomplete achromatopsia or severe, progressive cone dystrophy have also been reported. Rods continue to function because they express distinct CNG channel subunits. In contrast, null mutations in the rod CNG channel cause autosomal recessive retinitis pigmentosa (see review ), in which both rods and cones degenerate. Failure to express the CNG channel is lethal to rods and for reasons that are not yet known, death of the rods results in a secondary degeneration of cones so there is complete blindness rather than just night blindness.
The rod CNG channel allows many different cations to pass: Ca 2+ , Mg 2+ , Na + , even K + . Permeability is greatest for Ca 2+ , but under normal conditions, Na + carries ~80 percent of the current while Ca 2+ carries 15–20 percent , because the extracellular concentration of Na + is ten times higher than that of Ca 2+ . Mg 2+ influx comprises a few per cent of the circulating current. The proportion of current carried by Ca 2+ is twice greater through the cone channel because of its two-fold higher Ca 2+ to Na + permeability ratio. , Nevertheless, divalents are critical to the physiology of both rods and cones (see below and Chapters 18 and 20 ).
For fast responsivity to increases in [cGMP], it would be advantageous for the CNG channel to avidly bind cGMP. On the other hand, the channel would release cGMP too slowly and fail to detect decreases in cGMP rapidly. As a compromise, the channel has a fairly high K 0.5 for cGMP (i.e. moderately low sensitivity), on the order of 10 µM. , The channel subunits work together in such a way that cGMP binding affinity increases with each cGMP bound, making channel opening steeply dependent on ligand concentration ( Fig. 19.12B ). This cooperativity is one of Nature’s ways of building a biological switch. Cyclic GMP binding stabilizes the open state of the channel so by the law of microscopic reversibility, cGMP unbinds best from the closed state. Therefore, to be constantly vigilant for a decrease in [cGMP], the channel reverts incessantly to the closed state (see review ). The channel can then respond to changes in [cGMP] on a time-scale that is considerably faster than the photoresponse kinetics. Flickering makes the channel extremely noisy though ( Fig. 19.12C ). To minimize the noise, the channel detains Ca 2+ and Mg 2+ during their passage through the pore. As long as divalents reside in the pore, no other ions can pass and the conductance of the CNG channel drops by two orders of magnitude from about 25 pS to 100 fS. Given low channel conductance and relative insensitivity to cGMP, the cell must express a lot of channels (a few hundred per µm 2 ) in order to maintain a reasonable circulating current. The arrangement averages channel noise across many channels, but it also puts the cell at risk. Normally, the free [cGMP] in darkness is only a few micromolar and a small percentage of channels are open. Should the cell synthesize too much cGMP the number of open channels would increase by more than an order of magnitude and annihilate the transmembrane ion gradients.
For the purposes of photon counting, it would be desirable for the light-induced decrease in circulating current to be proportional to the number of channels closed. But CNG channel closure hyperpolarizes the rod and Ohm’s law dictates an increase in current flowing through each of the remaining open channels. Two mechanisms conspire against a linear Ohmic relation between current and voltage. First, hyperpolarization enhances divalent block by increasing the driving force on Ca 2+ and Mg 2+ to enter the channel pore. , Second, the CNG channel is slightly voltage dependent so hyperpolarization tends to close the channel. The net effect is that over the physiological range of membrane potentials, the inward current is nearly constant ( Fig. 19.13 ). The CNG channel in cones is also outwardly rectifying over the physiological range of voltages, but to a lesser extent than the rod channel. So in brighter light, as the cone becomes more hyperpolarized, the driving force sends more ions through the remaining open channels. Cones are not concerned with single photons and more interested in dynamic range. The reduced effect of closing channels at hyperpolarized potentials may contribute to their remarkable capacity to operate over a wide range of intensities. Another consequence is that the photocurrent in cones will not isolate events in the outer segment and can be influenced by voltage changes induced by external sources (see Chapters 21 and 22 ).
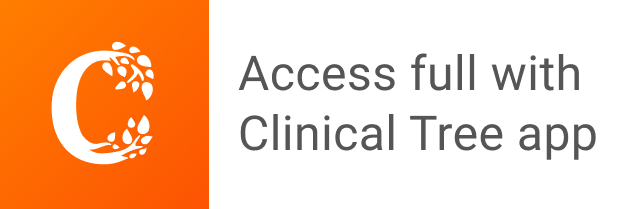