In the past decades, two significant changes have impacted how we contextualize the pathogenic mechanisms of primary open-angle glaucoma. The first change is clinically relevant: the elimination of intraocular pressure (IOP) from the essential definition of the disease. In other words, glaucomatous optic neuropathy is thought of as an optic nerve disorder in which IOP is one important causative, dose-related risk factor among several others.
The other important shift has been in the conceptual framework for pathogenesis. At one time, theories of glaucomatous pathophysiology were rhetorically confined to a coarse dichotomy, considered from either a ‘mechanical’ or a ‘vasogenic’ basis. Contemporary research has elucidated many intriguing and complementary details both from biomechanical and from vasogenic perspectives. And in addition, multiple insights into pathophysiology at the immunologic, cellular, and biochemical levels have begun to elucidate a variety of cascades, which together or separately may manifest in final pathways detectable to us as the clinical features of glaucomatous optic neuropathy.
All glaucomatous atrophy shares the following features : (1) progressive death of retinal ganglion cells, manifesting as (2) characteristic histopathologic alteration of the optic nerve – known as excavation – which is functionally apparent as (3) sequential visual field deterioration in characteristic patterns. Detailed understanding of the microanatomy of the optic nerve is intimately entwined with the current concepts of glaucomatous pathophysiology.
ANATOMY OF THE OPTIC NERVE HEAD
The optic nerve head (ONH) can be divided into four anatomic parts: the surface layer and the prelaminar, laminar, and retrolaminar portions. Each portion of the ONH is made up of axons (nerve fibers) grouped into bundles, blood vessels, and supporting glial tissue.
The superficial nerve fiber layer (SNFL) of the ONH has its most anterior limit at the point where the nerve contacts the vitreous. For histopathologic and clinical purposes, the peripheral edge of the nerve is defined by the anterior limits of the scleral ring. The posterior limit of the SNFL is recognized histologically as the point at which the axon bundles have completed their 90° turn from the plane of the retina and have reached the level of the choroid. The prelaminar portion of the ONH is the indistinct segment of the axons surrounded by the outer retina, choriocapillaris, and choroid; structurally the astroglial component here is considerably increased compared with the SNFL. The laminar portion of the nerve is contained within the lamina cribrosa; here the glial-wrapped axon bundles are confined in the relatively rigid pores of the specialized laminar scleral plates. Posterior to this is the retrolaminar portion of the optic nerve, where its thickness is doubled by the presence of myelinating oligodendrocytes. These and other eponymic details are illustrated in Figure 12-1 .

In the human eye the distribution of the nerve fibers from the peripheral retina toward the optic nerve is such that axons from peripheral ganglion cells are progressively overlayered by axons derived from cell bodies closer to the optic nerve ( Fig. 12–2 ). These peripheral fibers remain peripheral as they enter the disc; central fibers enter centrally, adjacent to the physiologic cup. This topographic arrangement correlates with the clinical progression of the glaucomatous visual field: paracentral scotomas appear early in the disease as the cup enlarges, and the peripheral field remains until the peripheral axons in the nerve are affected.


The arterial blood supply to the ONH varies among individuals, but there is general agreement about its fundamental components ( Fig. 12-3 ). The central retinal artery (CRA) and the short posterior ciliary arteries (SPCAs) all contribute directly or indirectly to a capillary plexus that supplies the ONH. The venous drainage of the ONH is almost entirely through branches of the central retinal vein, although important choroidal collaterals exist; these collaterals may appear as retinociliary shunts in instances of disturbed retinal circulation.

The branches of the CRA supply the SNFL. This is the network responsible for the flame- (splinter-) disc hemorrhages seen clinically, and it is also the vascular bed that appears in fluorescein angiograms of the ONH. The prelaminar ONH is supplied by branches of the SPCAs, which enter the disc substance through the adjacent sclera and posterior to the choroidal bed (see Figs 12-1 and 12-3 ). With one prolific exception, most investigators maintain that vessels derived from the peripapillary choroid make only a minor contribution to the blood supply of the anterior ONH.
The laminar portion is vascularized primarily by centripetal SPCAs, although an axial longitudinal anastomotic capillary bed has been described. The ability of that network to provide collateral circulatory support in the event of an arteriolar blockage, however, appears to be limited. The anterior portion of the retrolaminar nerve, however, enjoys both centripetal vascular supply from the pia-meninges and a significant axial vasculature from branches of the CRA.
MECHANISMS OF GLAUCOMATOUS OPTIC NEUROPATHY
A particularly cogent framework for integrating the vast amount of experimental and clinical observations of the various factors contributing to glaucomatous optic neuropathy has been elaborated by Quigley. His approach poses three queries: (1) What is the primary site of glaucomatous injury? (2) What factors contribute to ganglion/axonal injury? (3) Precisely how do the ganglion cells die? The merit of this scheme is that the multiple factors and pathogenic influences that have been elucidated can be accommodated without having to embrace the artificial, binary posturing of the mechanical versus vasogenic theories of causation.
WHERE ARE THE GANGLION CELLS INJURED?
There is consensus that the ONH itself – specifically the sclera lamina of the ONH – is a primary site of glaucomatous axonal injury. This has been observed in a variety of chronic glaucomatous experiments in primates and in enucleated human eyes with primary open-angle glaucoma (POAG). Histologically all orthograde and retrograde structures and systems of intra-axonal transport are disrupted in this laminar location of the axons’ course. Retrograde death occurs in the retinal ganglion cell body approximately 4 weeks later, and loss of the distal axon to the brain occurs within 1 week by Wallerian degeneration. Recent studies have also implicated both the venous outflow channels of the lamina cribrosa and the interplay of the retrolaminar cerebrospinal fluid pressure with the IOP in setting the translaminar tissue pressure. At a biochemical level, neurotoxic and glial-toxic nitric oxide enzymes have been localized to the ONH as well. Any theory of glaucomatous pathogenesis must therefore account for this site-specific localization to the lamina cribrosa for the earliest signs of axonal injury. Another promising line of research is also focusing on this laminar location, emphasizing its key anatomic position at the converging interface of four pressure compartments: the IOP, the retro-laminar sub-arachnoid space, the intracranial cerebrospinal fluid (CSF) space posteriorly, and the surrounding intra-orbital space. Alterations among trans-laminar pressure gradients, as with traumatic CSF leaks, may clinically contribute to glaucomatous progression.
Yet the inner retina is apparently another site of damage to both the retinal ganglion cell (RGC) and astroglial populations. It has been hypothesized that intraretinal or intravitreal glutamate levels that are neurotoxic to ganglion cells play a role in glaucoma. Such biochemical specifics suggest possible strategies of neuroprotective intervention. This approach is being actively pursued in other neurologic degenerative conditions such as Parkinson’s disease and Alzheimer’s disease although equivocal results in large studies caution enthusiasm for effective glaucoma interventions at this time.
The growing appreciation of astroglial roles in CNS neurotransmission significantly expands the scope for research into this heretofore overlooked cellular population’s possible role in neurodegenerative diseases, such as glaucoma.
Although the role of vascular perfusion remains relevant to elucidating glaucomatous pathophysiology, localization to specific vascular beds within the globe remains problemmatic. If generalized vascular insufficiency at the level of the retinal or choroidal circulations were primarily involved in glaucomatous optic atrophy, extensive deleterious effects on other retinal cell types would be expected, but this is not the case in the histopathology of glaucoma. Diffuse vascular insufficiency of an ocular layer, as is reportedly responsible for diffuse inner choroidal thinning in glaucoma, may contribute to the glaucomatous pathogenesis indirectly or in ways that remain unknown.
WHAT INJURES GANGLION CELLS?
This is a fascinating topic for which the impressive strides of recent glaucoma epidemiology bear directly on histopathologic events. For example, unequivocal clinical risk factors for developing glaucoma include IOP, increasing age, race, high myopia, family history of POAG, and a variety of vascular indexes. These factors should be distinguished from associated clinical findings that reflect early but established glaucomatous damage before manifesting as defects in the visual field. Such associated findings include large cup/disc ratio (and related indexes from ONH imaging); disc hemorrhages; nerve fiber layer defects; and abnormal psychophysical changes such as short-wavelength (blue-yellow) perimetric defects or altered contrast sensitivity.
Both risk factors and early alterations must necessarily be expressed at the cellular level. Quigley considers four aspects of potential ganglion cell injury.
Ganglion Cell Susceptibility
There are an average of one million ganglion cells per human eye, with tremendous variability by a factor of three to four. The size of the optic disc is a marker for axon number: the larger the scleral canal, the more nerve fibers are present. Interestingly, eyes with POAG do not have larger discs than do age-matched normals, and this also holds true for blacks who generally have larger optic discs than do whites. These data exemplify the paradoxic nature of many ‘susceptibility factors’: a larger optic disc would theoretically be more deformable and thus exacerbate axonal loss (see below); yet such large discs have more axons, whose greater numbers might reduce susceptibility to glaucomatous atrophy.
Aging takes a toll on the number of nerve cells throughout the brain, with a loss of approximately 25% over a lifetime, including loss of the retinal cell populations. Any acceleration of this process could manifest as clinical glaucoma. Elevated IOP, even in normal eyes, may be one such accelerant for subclinical axon loss. Thus increased age and elevated IOP may converge in hastening the natural attrition of ganglion cells.
Susceptibility may also reside in the specific subtype of ganglion cells. There are significant variations of ganglion cell populations among mammalian and primate eyes, with at least 13 varieties of RGCs reported in primates. The most commonly encountered ganglion cells in the human retina are small (parvo) cells and large (magno) cells, in a ratio of approximately 8:1, respectively. The parvo cells transmit acuity and color data; the magno cells convey motion perception and scotopic information.
The preferential loss of the magnocellular population in early glaucoma, reported by several investigators, may reflect one of several scenarios. Perhaps there is a genuine but unexplained intrinsic sensitivity of the magno cells to the (unknown) earliest toxic effects of glaucoma. A likelier explanation is that diffuse damage occurs to all axons in the ONH but manifests as an early magnocellular defect because there are fewer such fibers to begin with, and they tend to congregate in susceptible portions of the lamina cribrosa (see below). This is compatible with reports of early glaucoma damage to both parvocellular pathways (tested by short-wavelength perimetry) and magnocellular pathways (assessed by motion-automated perimetry). It is important to conceptualize the ‘redundancy’ or abundance of ganglion cells relative to actual clinical visual function. Approximately 25% RGC loss is required for an afferent papillary defect; approximately 35% RGC loss before defects are detected with computerized threshold white-on-white perimetry; and 40% RGC loss before acuity worsens. Clinical tests that can preferentially exploit the early loss of different ganglion cell populations and their functions are actively being pursued. (See Chapter 11 .)
Connective tissue structures within the optic nerve head
Because excavational cupping of the ONH is an essential characteristic of progressive glaucoma, the vulnerability and behavior of the structural elements of the optic nerve are a focus of intense interest. From a clinical perspective, the greater susceptibility of the myopic eye than the emmetropic or hyperopic eye to sustain glaucomatous damage suggests altered scleral rigidity or deformation of the posterior scleral structures as contributory factors. Research on the biomechanics of laminar and prelaminar tissues implicates the interplay of IOP and aging factors on the development of glaucomatous cupping.
Optic disc excavation is the consequence of three related events: (1) loss of neural rim axons; (2) elongation, stretching, and collapse of the laminar beams and their posterior axial displacement (bowing); and (3) outward, centrifugal rotation of the laminar insertion into the scleral insertion zone ( Fig. 12-4 ). Histopathologically, many cellular and subcellular alterations of the normal laminar structures have been associated with this distinctive form of optic atrophy. Changes include specific remodeling of the extracellular matrix of the laminar tissue and astrocytic reactivation, variations in elastin, suggestive of decreased compliance, and concomitant loss of the intralaminar microvasculature as the axonal mass is diminished. Combined, these various changes can result in an altered mechanical compliance of the ONH.




Another intriguing characteristic of the laminar architecture that bears directly on axonal injury in the ONH is the lower density of support in the upper and lower laminar pores, a finding seen in perhaps as many as 50% of glaucomatous eyes. The pores tend to be larger in the superior and inferior areas of the lamina cribrosa; this is thought to allow less support for, and more compressibility of, the arcuate axon bundles ( Fig. 12-5 ). Such vulnerability would result in their earlier loss in glaucoma in an hourglass configuration, manifesting as the arcuate visual field defects.


These regional anatomic differences of the lamina are the most dramatic demonstrable asymmetric structures that correlate with the clinical patterns of damage seen in glaucoma, but are not exclusive. For example, the finding of extremely thin lamina cribrosal structures in highly myopic eyes has led to the speculation that this could expose the ONH to steeper translaminar pressure gradients between the IOP and cerebrospinal fluid space, accounting for greater sensitivity to glaucomatous damage in such eyes. Variations in astroglial structures within the laminar region have also been reported.
Clearly the clinical risk factors of race and family history could be expressed as genetic aberrations in any one of the myriad cellular and synthetic processes alluded to above. Undiscovered alterations in the production or function of collagen, elastin, extracellular matrix, astrocytes, laminar architecture, or other structures in the ONH could result singly or jointly in the overall susceptibility of the ONH to excavational damage. Similarly, unknown genetic variations in ganglion cell populations, numbers, or sensitivities could also manifest as clinical disease.
Intraocular pressure level
Although it is unclear which cellular and histologic alterations are primary or secondary, elevation of the IOP is the most consistent and reproducible experimental model for producing pathognomonic glaucomatous excavation of the optic disc. Physicians have anecdotally observed excavational cupping, similar to that seen with glaucoma, in a variety of circumstances, including arteritic anterior ischemic optic neuropathy, compressive optic neuropathy, and optic nerve infarction. Under laboratory circumstances, however, neither optic nerve transection nor ischemic insults recapitulate the consistent ONH alterations seen both in prolonged experimental IOP elevation and in clinical glaucoma.
Precisely how IOP induces these unique changes remains unclear. Clinically it has been well established that there is a continuum of association between IOP and POAG, much as a dose-response curve. In actuality, there is no ‘normal’ IOP that serves as a fail-safe reading for determining the presence or absence of the risk or progression of glaucoma.
Some of the ambiguity surrounding the role of IOP is epidemiological: among Japanese or African-Americans, sensitivity to IOP levels for developing glaucomatous atrophy appears greater than in whites, although non-pressure factors, such as population-specific mean IOP values or the pressure-independent risk of central corneal thickness, may confound our understanding. Some of the ambiguity is technological: for example, pachymetric investigations of the effect of corneal thickness on applanation pressure readings suggest that even the categories of ‘ocular hypertensives’ and ‘low-tension glaucoma eyes’ may reflect instrument artifact, with thinner corneas reading lower IOPs and thicker corneas higher IOPs. Similarly, the intriguing possible relationship between IOP and the pressure gradient experienced by the ONH adjacent to the retrolaminar’s cerebrospinal fluid pressure remains to be elucidated experimentally and clinically.
Vascular nutrition of the optic disc
In the search for the other pathogenic factors involved in glaucomatous atrophy, either independent of or in concert with IOP, much has been published regarding the vascular status of the glaucomatous eye and patient. Some clinical reports have observed anecdotal worsening of glaucomatous field defects and the disc with therapeutic or pathologic reduction of the systemic blood pressure ; yet there is a contradictory study of glaucomatous patients who sustained severe hypotension without clinical deterioration. Epidemiologic assessments have revealed a complex relationship between systemic hypertension and glaucoma, involving age as a factor. High blood pressure is relatively protective against glaucoma in younger individuals; in older patients systemic hypertension is a risk factor. Conversely, a low diastolic blood pressure in conjunction with elevated IOP (average 26 mmHg) increases the glaucoma risk eight-fold.
Thus it may be particularly valuable to evaluate the synergy of risk factors such as blood pressure and IOP together, rather than approaching the problem from either a vasogenic or mechanical hypothesis of causation. In fact, retrospective multivariate analyses show complicated groupings of various factors.
Peripheral vasospasm has been found by some to be associated with low-tension (normal pressure) glaucoma. The hypothesis is that peripheral vasoconstriction, as measured in the finger’s nail-bed capillary responses to cold water immersion, correlates with altered endothelial autoregulation of the blood supply of the ONH. However, correlation with observable parameters such as peripapillary retinal arteriolar narrowing has not been clinically convincing. Similarly, other vasculopathic diseases such as migraine and diabetes mellitus have been both reported and reported not to be associated with glaucoma.
A fundamental issue of how vascular disorders could affect the ONH is the process of autoregulation . The body exercises various control mechanisms to maintain perfusion through myogenic and metabolic feedback loops. These include global control through cardiac output and blood pressure, local control for the regional distribution of flow, and hormonal control for prioritization of various vascular beds. Autoregulation refers to the initiation of a local response to control blood flow within a range of physiologic parameters. Such autoregulation is lacking in the choroid but present in both the retina and optic nerve. The extensive intraneural capillary bed in the ONH appears to be primarily responsible for this regulation. Deficient autoregulation has been reported both in the ONH and in the retina of glaucomatous eyes. The specific mechanisms of damage and response, however, remain elusive.
Another vascular phenomenon that has been extensively studied in glaucoma is fluorescein angiography of the disc. Focal areas of ‘filling defects’ can be seen in areas of excavation, but these are almost certainly non-specific, secondary changes due to the loss of capillaries in focal areas of optic nerve atrophy ( Figs 12-6 and 12-7 ). Moreover, disc angiograms record the vascular bed of the SNFL, derived from branches of the CRA. As such, little information about vascular events within the lamina cribrosa, where glaucomatous damage first manifests, is apparent.




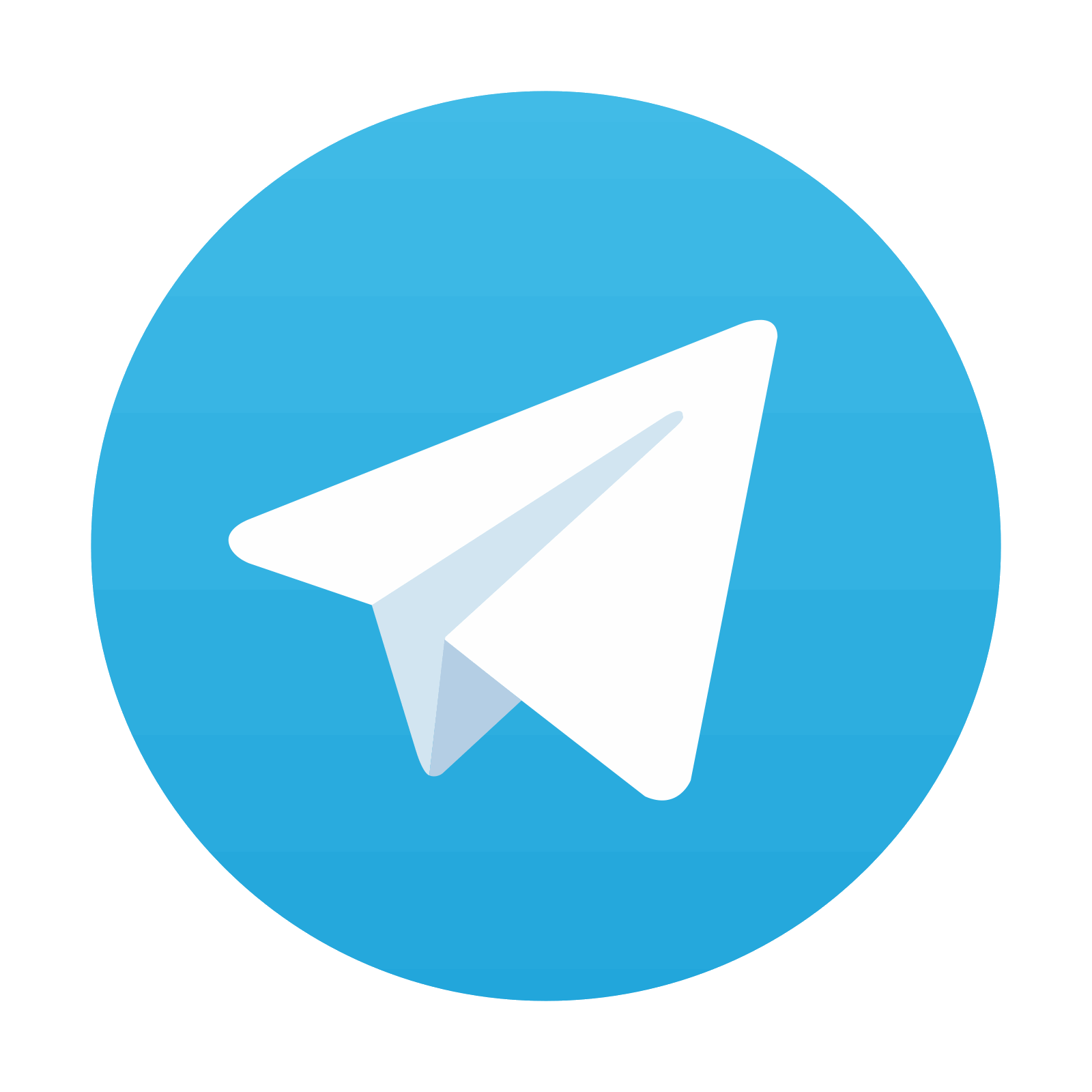
Stay updated, free articles. Join our Telegram channel
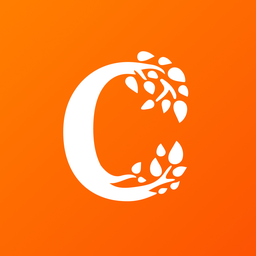
Full access? Get Clinical Tree
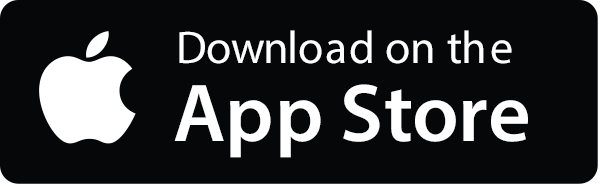
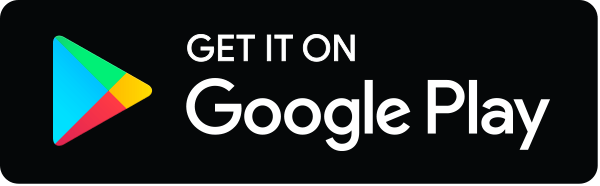
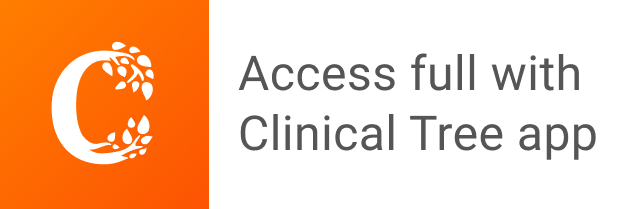