Introduction
The two optic nerves carry the axons of retinal ganglion cells (RGCs), and along these axons transmit all of the visual information from the inner retina ( Chapter 23 ) to the brain ( Chapter 29 , Chapter 30 , Chapter 31 ). Thus, diseases that affect the optic nerve commonly cause vision loss. The retina and optic nerve are developmentally an outgrowth of the forebrain, and, like other white matter tracts of the central nervous system (CNS), the optic nerve does not repair itself after most types of injury. Thus, blindness from optic nerve injury or degenerative disease is typically irreversible. This chapter reviews the principal aspects of optic nerve anatomy, development, and physiology, and discusses the pathologic changes at the molecular and cellular levels in the context of clinical disease.
Optic nerve anatomy
Retinal ganglion cell axons within the nerve fiber layer
RGC axons begin at the RGC bodies in the inner layer of the retina. Although most neurons in this layer are RGCs and the layer is commonly referred to as the ganglion cell layer , in humans approximately 3 percent of cells in the central retina and up to 80 percent in the peripheral retina may be other cell types, primarily displaced amacrine cells. In addition, studies in mammals have demonstrated a few displaced RGCs located in the inner nuclear layer. As discussed in Chapter 23 , the RGCs receive input from bipolar cells and amacrine cells, and project their axon towards the vitreous, whereupon they turn approximately 90° and project towards the optic nerve head in the nerve fiber layer . The nerve fiber layer is not quite radially arranged around the optic nerve head (optic disk), as axons near the center of our visual field course away from the fovea, and then towards the optic disk, entering in the superior and inferior portions of the disk ( Fig. 28.1 ). This interesting anatomy prevents axons from crossing the high-sensitivity fovea, where they might otherwise scatter light and degrade visual acuity. The axons from more peripheral RGCs are more superficial (vitread) to those arising from less peripheral ganglion cells ( Fig. 28.2 ). In addition, there is strict segregation of those fibers arising from RGCs located superior to the temporal horizontal meridian (raphe) and those fibers arising from RGCs located inferior to the horizontal raphe. Because of this segregation, visual field defects corresponding to injury to RGC axons typically have stereotyped patterns, e.g. superior or inferior nasal steps, temporal wedges, or arcuate scotomas. These are called nerve fiber bundle defects, and with other visual field defects are covered in more detail in Chapter 35 . The nerve fiber layer can be measured using various modalities in human eyes ( Box 28.1 ).


The number of RGC axons in the optic nerve is, in most cases, probably well-approximated by the number of RGC axons in the nerve fiber layer of the retina immediately adjacent to the optic nerve. The peripapillary nerve fiber layer can be measured quantitatively using a variety of modalities including coherence tomography (OCT), which measures layer thickness based on reflectance of light from the interfaces between layers, and by scanning laser polarimetry (SLP), which estimates nerve fiber layer thickness based on the interaction of polarized light with organized bundles of microtubules inside axons. Each of these technologies may face limitations. OCT estimation of RGC axons may be complicated by the presence of retinal astrocytes, which accompany RGC axons through the nerve fiber layer and contribute to nerve fiber layer thickness. SLP estimation of axon integrity may be limited by degradation or disorganization of RGC microtubules that may precede axon loss. All such technologies are limited by their inability to measure optic nerve axons specifically, and rather substitute peripapillary axon measurements.
Intrascleral optic nerve
The optic nerve itself is considered to begin at the optic nerve head which, when viewed through the front of the eye, is observed as the optic disk . There is an approximately 1-mm component of optic nerve within the intrascleral part of the globe, which includes the lamina cribrosa ( Fig. 28.3 ). Once at the optic disk, ganglion cell axons turn away from the vitreous and dive into the optic disk towards the brain. Axons arising from more peripheral RGCs are peripheral within the optic nerve head. The optic disk contains the nerve fibers around its edge, the neuroretinal rim , and the central cup which does not contain RGC axons. The cup-to-disk ratio may range from 0 to 1.0, depending on natural variation in the size of the disk, and whether there is less than a full complement of axon fibers, for example as a result of damage from glaucomatous optic neuropathy (see below).

Intraorbital optic nerve
The optic nerve extends approximately 30 mm from the globe to the optic canal. The straight-line distance from the back of the globe to the optic canal is much less (the exact amount depending on individual orbital depth), with the excess optic nerve laxity allowing for free movement of the globe during eye movements. Excessive proptosis stretches the optic nerve tautly, resulting in some cases in direct injury to or even avulsion of the nerve itself. Beginning behind the globe, the nerve is ensheathed by the layered meninges extending from the brain, bathing the optic nerve in cerebrospinal fluid and providing vascular support along the length of the nerve (see below).
The retinotopic segregation of RGC axons (topographic correspondence of optic nerve fibers to retinal location), particularly the segregation between axons arising from the superior and inferior retina, is gradually lost as the axons course through the nerve ( Fig. 28.3 ). There is only moderate retinotopy in the initial segment of the optic nerve. The retinotopy decreases distally, and then becomes ordered vertically for eventual nasal decussation near the chiasm. The loss of retinotopy is not absolute, because the fibers from the nasal (but not temporal) macula continue to be located centrally within the nerve over a considerable distance. Fibers from large RGCs are less retinotopically organized than those from smaller ganglion cells. Although studies in humans are difficult to do ante-mortem, post-mortem studies of nerves with specific visual field defects demonstrate similar findings, while post-mortem studies of developing fetuses and adult eyes also show loss of retinotopy within the transition from the optic nerve head to the nerve. Within the orbit, the optic nerve travels within the muscle cone formed by the superior rectus, lateral rectus, inferior rectus, and medial rectus muscles. Tumors within the cone are common sources of compression of the optic nerve, or compressive optic neuropathy . Examples of these tumors include cavernous hemangioma, hemangiopericytoma, fibrous histiocytoma, lymphoma, and schwannoma. In addition, enlargement of the muscles themselves, particularly the inferior rectus and/or the medial rectus in Grave’s ophthalmopathy, may also compress the optic nerve.
The optic canal
The optic nerve enters the cranium via the optic canal, a 5–12 mm passage which lies immediately superonasal to the superior orbital fissure. The optic canal contains some axons of sympathetic neurons destined for the orbit, as well as the ophthalmic artery. The latter lies immediately inferolateral to the optic nerve itself, covered in dura. At the distal end of the canal, there is a half-moon-shaped segment of dura which overhangs the optic nerve superiorly, and thereby lengthens the canal by a few millimeters. As in the intraorbital portion of the optic nerve, within the canal, and immediately posterior to the optic canal, meningeal tissue ensheathes the optic nerve. Benign tumors of the meninges, or meningiomas, are frequent causes of compressive optic neuropathies in these locations. Small tumors within the canal itself, where there is very little free space, may lead to compressive optic neuropathy without a radiographically visible tumor.
Intracranial optic nerve and the optic chiasm
Once the nerve has entered the cranium, there is a highly variable (8–19 mm, mean of 12 mm) length of nerve until the chiasm is reached. The length of the chiasm itself is approximately 8 mm. The intracranial optic nerve and chiasm are immediately above the planum sphenoidale and sella turcica, the latter of which contains pituitary gland. There is approximately 10 mm between the inferior part of the nerve and the superior part of the pituitary. Tumors of the pituitary which increase in size enough to compress the chiasm may therefore cause compressive optic neuropathy.
At the optic chiasm, RGC axons from the temporal retina remain ipsilateral, and those from the nasal retina cross the chiasm and course towards the contralateral brain. The ratio of fibers which cross versus those which do not cross is anatomically 53 : 47 and functionally 52 : 48. This small difference between the number of crossing and non-crossing fibers is commonly reported to be responsible for the relative afferent pupillary defect seen in disorders of the optic tract, in which an afferent pupillary defect is seen contralateral to the injured tract (see Chapter 25 ), but could also reflect the fact that some fibers from specialized cells within the retinal responsible for the pupillary reflex may cross from the temporal retina into the contralateral optic tract.
The optic tract and lateral geniculate nucleus
Although the optic nerve anatomically ends at the chiasm, RGC axons continue within the optic tract until the lateral geniculate nucleus, superior colliculus, pretectal nuclei, or hypothalamus (see below). Circuitry and processing by these targets are discussed in Chapter 25 , Chapter 29 .
Optic nerve axon counts and dimensions
In the normal adult human optic nerve, manual techniques have demonstrated an estimated 1,200,000 RGC axons per nerve; automated counting algorithms give figures between 700,000 and 1,400,000 axons. There is a strong correlation between the size of the neuroretinal rim and the number of axons, and between the number of axons and the size of the scleral canal in primates, although the degree of correlation is controversial.
Many factors affect the number of axons within the optic nerve, from inherited differences to damage from disease, i.e. optic neuropathy (see below). In addition, there is a gradual loss of RGCs during normal human aging, with an approximate 5000 axon loss per year of life. For unclear reasons, there is a smaller degree of loss of macular RGCs with age, compared to peripheral RGCs, and this may reflect contraction of the macula with time.
Although the number of RGC axons entering the optic nerve is fairly constant, the diameter of the optic nerve varies widely. At the disk itself, where the fibers are completely unmyelinated, the mean vertical diameter of the disk is 1.9 mm (range 1.0–3.0 mm) and the mean horizontal diameter is 1.7–1.8 mm (range 0.9–2.6 mm). The mean area of the disk is 2.7 mm 2 (range 0.8–5.5 mm 2 ). The mean area of the neuroretinal rim (not including the cup) is 2.0 mm 2 (range 0.8–4.7 mm 2 ). Because the axonal tissue entering the optic disk varies much less than the size of the optic disk itself, the optic cup in the center of the disk can vary greatly without necessarily reflecting any underlying deficit in the number of ganglion cell axons. The diameter of the optic nerve approximately doubles posterior to the globe as a result of myelination of the axons.
Microscopic anatomy and cytology
Axons
There are no other neuronal cell bodies within the optic nerve, making it a pure white matter tract of the central nervous system. Although the optic nerve itself may contain other small nerves, particularly tiny peripheral nerves (branches of the trigeminal system) which carry pain sensation or control vascular tone, the vast majority of the optic nerve is composed of the approximately 1,200,000 axons of the retinal ganglion cells. Optic nerve axons are collected in fascicles, which are separated by pia-derived septa. The number of fascicles ranges from approximately 50 to 300, being maximal immediately retrobulbar and at the optic canal. The mean axon diameter is slightly less than 1 µm, with a unimodal distribution skewed to the left. The mean diameter may decrease during aging, either through loss of large-diameter axons, or from a general, aging-induced atrophy. Compared with the optic tract, there is relatively little segregation of axons by size within the optic nerve, except for a tendency for finer axons to be located inferocentrotemporally.
There is variability of axonal diameter and myelin thickness from the retina to the brain. Studies in the ferret show that the diameters of the largest axons increase as the distance from the retina increases. The diameter of individual axons is regulated by multiple factors, including oligodendrocytes and activity, increases during development, and correlates with local accumulation of specifically phosphorylated neurofilament proteins.
Oligodendrocytes and myelin
Conduction of nerve impulses down the axons in the optic nerve depends on the presence of myelin, a fatty multilaminated structure which insulates each axon and greatly increases the speed and efficiency of conduction (discussed below). The retrolaminar optic nerve is completely myelinated under non-pathological circumstances ( Fig. 28.4 ). There are non-myelinated axons of the peripheral nervous systems within the adventitia of the central retinal artery and non-myelinated and myelinated Schwann cells around peripheral axons of the outer dura. Each axon is myelinated with several lamellae of myelin bilayers, with the number of lamellae varying from axon to axon but in strict proportion to the diameter of the axon. Individual oligodendrocytes elaborate an average of 20–30 processes per cell, each of which myelinates 150–200 µm of axon length.

Oligodendrocytes and axons regulate each other during development and throughout adulthood. Oligodendrocytes depend on the presence of axons for their survival, and for production of myelin proteins. Axons regulate oligodendrocyte survival and proliferation, and thus their number, through expression of specific signaling proteins and through axonal electrical activity, controlling oligodendrocyte number to match the number of axons. Conversely, axons are signaled by oligodendrocytes to regulate axon number and diameter, and to prevent axon sprouting or branching in the optic nerve. Later, in the adult, oligodendrocytes and myelin are partially responsible for inhibition of axon regeneration after optic nerve damage or degeneration (see below).
Astrocytes
Astrocytes, named for their stellate appearance, are ubiquitous glial components of the central nervous system, and function in white matter tracts like the optic nerve to regulate ionic and energy homeostasis. Astrocytes are highly efficient at transporting potassium, and increases in the level of extracellular potassium as a result of repolarization are buffered by astrocytes. Their ability to accumulate glycogen may allow them to serve as an energy source for the optic nerve in the absence of glucose (e.g. ischemia), by shuttling lactate to adjacent axons. Astrocytes form the glial-limiting membrane, and their processes are concentrated at the nodes of Ranvier and in contact with nearby capillaries. This positions the astrocyte to play a role in transportation of substances between the local circulation and the axons; in inducing endothelial cells to form the blood–brain barrier; and perhaps in signaling blood vessels to dilate or constrict according to local metabolic needs. Astrocytes also mediate connectivity between optic nerve axons and the adjacent connective tissues, such as the pial septa, the adventitia of the central retinal artery and vein, and the pia in a layer named the glial mantle of Fuchs.
Interestingly, the most common intrinsic tumor of the optic nerve is an astrocytoma, or optic nerve glioma. This is usually a low-grade tumor of well-differentiated astrocytic cells that appear hair-like, or “pilocytic.” Pilocytic astrocytomas are usually seen in childhood, and have a favorable prognosis. They are also commonly seen in association with neurofibromatosis. Rarely, more malignant neoplasms of astrocytic origin develop in adults. These resemble the higher-grade astrocytic neoplasms found elsewhere in the central nervous system, and are usually fatal.
Microglia
Microglia, a type of resident macrophage, are an important cellular component of the optic nerve. Although their origin was debated for decades, they have been shown experimentally to be of peripheral, bone marrow origin, and are not derived from the neuroectoderm that yields neurons, astrocytes, and oligodendrocytes. Microglia share several markers with macrophages, with both having Fc receptors (for immunoglobulin), C3 receptors (for complement), binding of Griffonia isolectin B4, and antigenicity for F4/80 and ED1 monoclonal antibodies. In the human optic nerve, microglia can be seen at 8 weeks after conception, when they are relatively undifferentiated. Microglia become more differentiated during fetal development, going from tuberous to amoeboid to a ramified morphology. They are associated with axon bundles, but not necessarily with blood vessels, and are found in both the nerve parenchyma and its meninges. Nerve and meningeal microglia are similar ultrastructurally except for vacuoles and endoplasmic reticulum in the former. This may be due to their phagocytosis of dying axons during development. Microglia share several characteristics of immune capacities of macrophages. By phagocytosing extracellular material, degradation within intracellular compartments can occur. This may be followed by antigen presentation on the cell surface. In combination with certain histocompatibility antigens, this presented antigen can cause stimulation of T lymphocytes and subsequent immune system activation (see below).
Meninges and meningothelial cells
The optic nerve is covered with three layers of meninges, dura, arachnoid, and pia. The meninges can also be divided up into pachymeninges (dura) and leptomeninges (arachnoid and pia). The outermost dura is a thick fibrovascular tissue, which is in immediate contiguity with the sclera, periorbita, and the dural layer of the lining of the cranial contents. The middle arachnoid layer is a loose, thin, fibrovascular tissue. The innermost pia is a thin, tightly adherent layer with extensions into the nerve itself forming the pial septae, through which the fascicles of ganglion cell axons course. In the optic canal, there are numerous trabeculae connecting the dura through the arachnoid to the pia, which reduce the free space of the nerve sheath in this area.
The space between the dura and arachnoid is the subdural space, while the space between the arachnoid and pia is the subarachnoid space. The subdural space around the optic nerve is small, and is not in communication with the intracranial subdural space. The subarachnoid space, on the other hand, is in communication with the intracranial subarachnoid space. The optic nerve subarachnoid space ends anteriorly within a blind pouch just before the optic disk. This extension of the subarachnoid space from the brain into the orbit explains why elevated intracranial pressure may cause compression of the optic nerve via elevating the hydraulic pressure. There is an appreciable pressure within the subarachnoid space, measuring from 4 to 14 mmHg.
Meningothelial cells may have several functions, including wound repair and scarring, phagocytosis, and collagen production. The meningeal vessels include pericytes and endothelial cells, which form tight junctions. Macrophages and mast cells are also found in the meninges. The role of these and other resident cells in meningeal inflammations is only speculative.
Blood supply
Optic nerve head
Studies of histological sections and of corrosion casting of the vessels themselves have added greatly to our understanding of the optic nerve blood supply ( Fig. 28.5 ). The ophthalmic artery provides the major vascular supply to the inner retina and optic nerve. The central retinal artery branches off of the intraorbital ophthalmic artery, and enters the optic nerve during approximately 12 mm behind the globe. In the retina, RGC bodies and the nerve fiber layer are supplied by capillary branches derived primarily from the central retinal artery arising out of the optic nerve head. As it branches on the optic disk into the retinal arterioles, the central retinal artery provides partial perfusion of the superficial optic disk via small capillaries. Along the optic nerve itself, however, the central retinal artery provides minimal perfusion of the nerve through which it courses.

In contrast, branches of the medial and lateral short posterior ciliary arteries, originating from the ophthalmic artery, provide the major blood supply to the optic nerve head, as well as the choroid. Their branches perfuse the optic nerve, both anteriorly via direct branches and posteriorly via a retrograde arteriolar investiture of the optic nerve (see below). Anastomoses of posterior ciliary artery branches form the circle of Zinn-Haller, which contributes significant perfusion to the optic nerve head. In addition, there are contributions from recurrent choroidal arterioles to the prelaminar and laminar optic nerve head, and contributions from recurrent pial arterioles to the laminar and retrolaminar optic nerve head. A clinical implication of the common posterior ciliary arterial source of the choroid and deep optic nerve head is that they will fluoresce simultaneously during the earliest phase of fluorescein angiography, before the retinal arterioles transit dye.
Unlike the choroidal vessels, which have fenestrated endothelial cells, optic nerve vessels have non-fenestrated endothelial cells with tight junctions, surrounded by pericytes. Optic nerve vessels therefore share the same blood–nerve barrier characteristics as the blood–brain barrier. Only a restricted number of molecules can cross the blood–nerve barrier. For example, gadolinium enhancement on magnetic resonance imaging is not seen unless there is some pathological process within the optic nerve that would disrupt the blood–nerve barrier, e.g. inflammation. Another major difference between choroidal and optic nerve head vessels is that only the latter can autoregulate, i.e. maintain approximately constant blood flow despite most changes in intravascular or intraocular pressure. Thus there is compensation of perfusion for a wide range of intraocular pressures in normal individuals. A dysregulation in optic nerve head autoregulation, and any ischemia that would follow, may contribute to the pathophysiology of glaucoma (see below).
Intraorbital optic nerve and optic canal
The intraorbital optic nerve is perfused primarily by the pial circulation, which branches off of the ophthalmic artery either directly or indirectly via recurrent branches of the short posterior ciliary arteries. The pia sends penetrating vessels into the intraorbital optic nerve along the fibrovascular pial septae, from which a capillary network extends into neural tissue (axons and glia). Similarly, the intracanalicular optic nerve is perfused by up to three branches of the ophthalmic artery, namely a medial collateral, lateral collateral, and ventral branch, which perfuse the pial surface and then penetrate the nerve. An important clinical implication of the pial supply relates to optic nerve sheath meningiomas. If a surgeon strips the meningioma away from the nerve, then the pia will be removed as well, resulting in loss of the blood supply to the nerve, and possible infarction and blindness. Another clinical correlation is related to the small amount of free space within the canal, when occupied by blood from shearing of the delicate vessels there, this may result in a sight-threatening compressive hematoma (and see below).
Intracranial optic nerve, chiasm, and optic tract
The intracranial optic nerve and chiasm are perfused by the internal carotid artery and its branches, primarily the anterior cerebral, anterior communicating, and the superior hypophyseal artery. The posterior chiasm may also be perfused by branches of the posterior communicating artery. The optic tract is predominantly perfused by branches of the posterior communicating and anterior choroidal arteries. Similar to the intraorbital and intracanalicular optic nerve, the blood supply occurs via small pial penetrating vessels. However, there is no dura or arachnoid surrounding the optic nerve posterior to the optic canal, and thus the risk of infarction is not from stripping a tumor off the nerve, but from inadvertent detachment of the fine vessels during surgical manipulation.
Vascular biology
Vessels of the human optic nerve contain endothelial cells separated from pericytes by a basement membrane, with endfeet of astrocytes surrounding the two. The tight junctions between endothelial cells contribute to the blood–nerve barrier, which is present in normal optic nerve throughout its length. The anterior part of the optic nerve, however, shares a distinctive feature with the area postrema, choroid plexus, and median eminence, in that there is local disturbance of this blood–brain barrier. Although the optic nerve capillary endothelial cells in the anterior optic nerve have tight junctions, leakage can occur from adjacent choriocapillaris through the border tissue of Elschnig at the level of the lamina choroidalis. Further leakage from this area into the subretinal space may be prevented by tight junctions between the glia making up the intermediary tissue of Kuhnt. The blood–nerve barrier may be incompetent in focal inflammatory disorders, such as optic neuritis.
The optic nerve differs from almost all other areas of the CNS in that there is connective tissue around vessels. The vessels are closely surrounded by morphologically specialized collagen and pial fibroblasts, beyond which are astrocytic foot processes. These pial septae are not a constant feature of mammalian optic nerves; rats, for example, do not have septae.
The vessels within the nerve have their own innervation. These nervi vasorum contain multiple neurotransmitters including adrenergic, cholinergic, and calcitonin gene-related peptide; neuropeptide Y; substance P; and vasoactive intestinal peptide. Human posterior ciliary arteries respond to the potent vasoconstrictor angiotensin II.
Optic nerve development
Generation of optic nerve oligodendrocytes and myelination
Oligodendrocytes develop from oligodendrocyte precursor cells (OPCs). OPCs migrate from the brain into the optic nerve, perhaps from the base of the preoptic recess, and this migration may continue during adult life. The OPC has a complex regulatory mechanism, with in vitro and animal studies implicating platelet-derived growth factor (PDGF), basic fibroblast growth factor (bFGF), ciliary neurotrophic factor (CNTF), and other factors in the control of differentiation, division, and renewal of the progenitor cell population. An intrinsic developmental clock controls the differentiation of OPCs into oligodendrocytes, after which the cell is mitotically unresponsive to PDGF. In normal development, some OPCs persist into the adult and may participate in remyelination after injury or in diseases like optic neuritis.
After oligodendrocyte differentiation from OPCs, there is typically a short delay before myelination starts, which may be regulated by inhibitory signals from axons activating the notch signaling pathway. The developmental regulation of myelination of the optic nerve and tract occurs earliest at the brain end of the optic nerve, then travels distally toward the eye, i.e. proximally with respect to the ganglion cell axon. This is opposite to myelination elsewhere in the CNS, which usually occurs from the neuron cell body outward along the axon. The brain-to-eye direction of myelination correlates with animal studies demonstrating OPC migration and then differentiation outward along the optic nerve. In humans, oligodendrocytes are not seen in the optic nerve before 18 weeks of gestation. Later, optic tract and intracranial nerve axons begin myelination at 32 weeks of gestation, with the process virtually complete at birth. The axons adjacent to the globe begin myelination only at birth; all are myelinated by about 7 months of age. Further myelination continues, however, and thickening of axonal myelin may continue past 2 years, or longer.
In longitudinal studies of rat optic nerve development, axons that become myelinated increase in diameter. The signal for change in caliber is generated by the oligodendrocytes. Although unmyelinated and not yet myelinated axons can conduct electrical impulses in the developing optic nerve, there is a surprising increase in conduction velocity during development, independent of diameter or myelination status, the reasons for which are unclear.
Interestingly, intraretinal RGC axons are not normally myelinated in most species; indeed, there are normally no oligodendrocytes in the retina. Studies of rat optic nerve head and anterior optic nerve demonstrate an abnormal transition zone from unmyelinated axons to myelinated axons, with varying lengths and thicknesses of myelin, unusual nodal region morphology, and few normal oligodendrocytes. Studies of rat optic nerve suggest that a signal found at the lamina cribrosa blocks the migration of OPCs into the retina. This barrier is possibly due to the presence of specialized optic nerve head astrocytes, serum factors from a focally deficient blood–nerve barrier or the adhesion substrate tenascin. In rabbits, which have no lamina cribrosa, there are axons myelinated by oligodendrocytes in the retina, and intraretinal OPCs can be identified. Further, the retinal portion of the ganglion cell axon has no constitutive barrier to myelination because ganglion cell axons of retina transplanted into rat midbrain can be myelinated, and OPCs injected intraretinally can differentiate and myelinate RGC axons. Myelination may also be artifactually induced by injuring the retina from the scleral side. This trauma leads to migration of Schwann cells and subsequent intraretinal myelination. In human eyes, myelination of part of the nerve fiber layer is occasionally seen; it is assumed that this is secondary to ectopic oligodendrocytes, although there are reports of Schwann cells myelinating intraretinal axons in the cat and rat. Although oligodendrogliomas within other parts of the central nervous systems are sometimes seen, neoplasms of oligodendrocytes within the optic nerve are very rare.
Generation of optic nerve astrocytes
Astrocyte precursor cells have been considerably less well studied. Like oligodendrocytes, developmental regulation of astrocyte number is determined by the RGC. Astrocyte precursor cells may depend on CNTF or LIF for differentiation into astrocytes, at which point they upregulate the expression of glial fibrillary acidic protein (GFAP), an intermediate filament protein. GFAP itself is important to optic nerve development, as transgenic mice lacking GFAP demonstrate abnormal myelination and blood–brain barriers. Astrocytes may also be critical for optic nerve development in that they may serve as the substrate over which extending ganglion cell axons grow.
Development of optic nerve meninges
The anatomy and development of the optic nerve meninges have been well described. All three meningeal layers are formed from fibroblast-like cells, which vary in ultrastructural appearance from typical process-bearing fibroblasts to mesothelial cells lining the subdural and subarachnoid space. The meningeal layers are particularly rich in collagen and elastin. Trabeculae between the arachnoid and pia are formed from collagen and are lined with the same mesothelial cells that line the subarachnoid space. The most rapid proliferation of human meningeal cells during development occurs within 8–18 weeks of conception, with centrally located fibroblasts and peripherally located cells containing glycogen that increase during this time. By 14 weeks, the three layers of the meninges can be distinguished. These glycogen-rich cells go on to produce large amounts of collagen, which is deposited in the dura after 14 or 15 weeks.
Axon number
Studies in animals suggest that approximately 50–100 percent excess RGCs are produced in the retina. During early development, the number of RGCs decreases by programmed cell death called apoptosis (discussed in the context of axon injury, below), in many cases because not all of the axons properly connect to their target regions within the brain. This form of programmed cell death may be adaptive if it does not release toxic intracellular components into the extracellular environment and thereby does not affect neighboring cells that are already integrated to survive. Likewise, in humans, up to two-thirds of developing RGCs die, predominantly during the second trimester.
Axon growth
Considerable progress has been made in recent years in our understanding of the development of the optic nerve, particularly at the level of molecular and cellular regulation. Axon growth requires specific extracellular signals – merely inhibiting cell death is not sufficient for inducing axon growth in developing RGCs. The most potent signals for axon growth are the same peptide trophic factors that most strongly promote RGC survival, and multiple trophic factors can often combine to induce even greater axon growth. The best-studied peptide trophic factors for RGC axon growth are brain derived neurotrophic factor (BDNF), ciliary neurotrophic factor (CNTF), insulin-like growth factor (IGF), basic fibroblast growth factor (bFGF), and glial cell line-derived neurotrophic factor (GDNF), although it is not clear how critical any of these are for axon growth during normal development. Other than peptide trophic factors, extracellular matrix molecules like laminin and heparin sulfate proteoglycans, and cell adhesion molecules like L1 and N-cadherin are expressed in the visual pathway and provide a critical substrate along which axons extend. Eliminating N-cadherin expression, for example, clearly decreases RGC axon growth in vivo. Finally, other extracellular signals as simple as purine nucleotides may be critical to induce axon growth, although the mechanism of this activity is still mysterious.
Electrical activity (discussed below) is not required for axon growth, but likely plays a role in sculpting axonal morphology. Thus, after injecting tetrodotoxin into the eye to block action potentials, or in experimental mice in which synaptic release of neurotransmitters is essentially eliminated, RGCs and other CNS neurons successfully elongate their axons to their targets in the absence of electrical activity. RGC activity may increase the rate of axon arborization in their target fields by stabilizing growing branches, and may influence the specificity of local connectivity and targeting of axons. Considerable progress has been made in understanding these molecular mechanisms for axon growth, and these have been reviewed elsewhere.
Axon guidance
Optic nerve axon guidance is heavily influenced by the earliest pathfinding axons, which serve as a substrate and guidance cue for the growth of the axons that follow. During development, RGC axons are guided by neuronal and glial molecules that attract and repel axonal growth cones. For example, embryonic RGC axons are repelled by Müller glia away from deeper retinal layers into the nerve fiber layer. Within the retina, the interesting course of retinal axons in the macular nerve fiber layer are likely due to a foveal repellant combined with an optic disk attractant. RGC axons are directed centrally in the retina away from chondroitin sulfate proteoglycans (CSPGs) and are attracted into the optic nerve head by netrins. A semaphorin, sema5A, may contribute to keeping the developing axons within the substance of the developing optic nerve. Ipsilateral-projecting axons are repelled at the optic chiasm by slit1 and slit2, ephrin-B, and CSPGs. The developmental ordering of the axons as they pass through the chiasm and go on to innervate the lateral geniculate primarily reflects their time of arrival.
RGC axons reach four main targets: (1) the lateral geniculate nucleus of the thalamus; (2) the superior colliculus; (3) the pretectal nucleus; and (4) the suprachiasmatic nucleus. Most axons course unbranched to the lateral geniculate nucleus, but studies in many animals, including primates, have demonstrated the existence of axon collaterals to the other targets, suggesting that the same may be true for human optic nerves.
The lateral geniculate nucleus is the primary synaptic target for visual information processing on the way to the visual cortex (discussed at length in Chapter 29 ). The primate lateral geniculate nucleus has six layers: layers 1 and 2 consist of large (magnocellular) cells, and layers 3, 4, 5, and 6 consist of small (parvocellular) cells. The magnocellular layers receive input from the midget RGCs, while the parvocellular layers receive input from the parasol RGCs. Layers 2, 3, and 5 receive input from the ipsilateral retina, while layers 1, 4, and 6 receive input from the contralateral retina. In addition, there are interlaminar cells forming the koniocellular layers. These cells are smaller than those of the parvocellular layers, and receive synaptic input from blue-yellow RGCs.
A second set of optic nerve axons project to the superior colliculus, a paired structure of the dorsal midbrain. In lower animals, e.g. rodents, the majority of fibers of the optic nerve project to the superior colliculus. In primates, including humans, only a minority does so, with the majority of axons destined instead for the lateral geniculate nucleus. These fibers arise from most of the retina, except for the central 10 degrees. The superior colliculus has a laminar organization, and is retinotopically mapped. Most studies of superior colliculus architecture have been done in animals, although the human superior colliculus appears to have similar anatomy. The function of the superior colliculus is to coordinate retinal and cortical control of saccades and fixation. Fibers branching to midbrain terminal nuclei stabilize the retinal image during movement of the eye, head, or body. The role of the superior colliculus in extraocular movements is covered in Chapter 9 .
The pretectal nucleus in the midbrain receives RGC fibers important for the pupillary response. The pretectal projection is bilateral, and in addition fibers from each pretectal nucleus project both ipsilaterally and contralaterally to the Edinger–Westphal subnuclei of the third nerve nucleus. The latter then projects parasympathetic axons along the course of the third nerve to the ciliary ganglion within the orbit, where a synapse is made. Postsynaptic parasympathetic fibers continue to the pupilloconstrictor muscle, constriction of which is visible as the pupillary light reflex. The combined ipsilateral and contralateral projections from the retina to the pretectal nuclei, and from the pretectal nuclei to the Edinger–Westphal subnuclei necessitate that a light stimulus causing impulses along either optic nerve will cause equal constriction of both pupils. This is the physiological basis for detecting an afferent pupillary defect (also called a Marcus Gunn pupil). If there is a functional difference in the number of optic nerve axons carrying impulses from each retina, then light shined into the eye with fewer axons will cause a lesser amount of (bilaterally symmetric) pupillary constriction than light shined into the other eye. By alternating the light between the two eyes and observing the difference in pupil size dependent on which eye the light is shined into, one can detect which optic nerve is functionally conducting less than the other. This measure is highly correlated with differences in the number of surviving RGCs or RGC axons. It has been proposed that there are differences between the myelin surrounding axons destined for the lateral geniculate nucleus and those responsible for the pupillomotor reflex because of the existence, albeit rare, of patients with demyelinative optic neuropathies and monocular blindness with preserved pupillary reflexes. These patients do not have apparent conduction toward the lateral geniculate nucleus but do have conduction toward the pretectal areas responsible for the pupillary reflex. Detection of the afferent pupillary defect is discussed at length in Chapter 25 .
A fourth projection of optic nerve axons is via the retinohypothalamic tract to the suprachiasmatic nucleus and the paraventricular nucleus within the hypothalamus. These fibers are responsible for circadian control of the sleep–wake cycle, temperature, and other systemic functions.
Retrograde labeling studies in pigeons and rats indicate that only a few percent of RGC axons project to the pretectal and suprachiasmatic nuclei. Recently, a population of intrinsically photosensitive RGCs has been identified, that express the photopigment melanopsin, and project to the subthalamic nuclei to mediate circadian rhythm and pupillary light response. Thus, even if all rod and cone photoreceptors are lost (as in retinitis pigmentosa, for example), these intrinsically photosensitive RGCs can continue to collect light for circadian rhythm entrainment, for example.
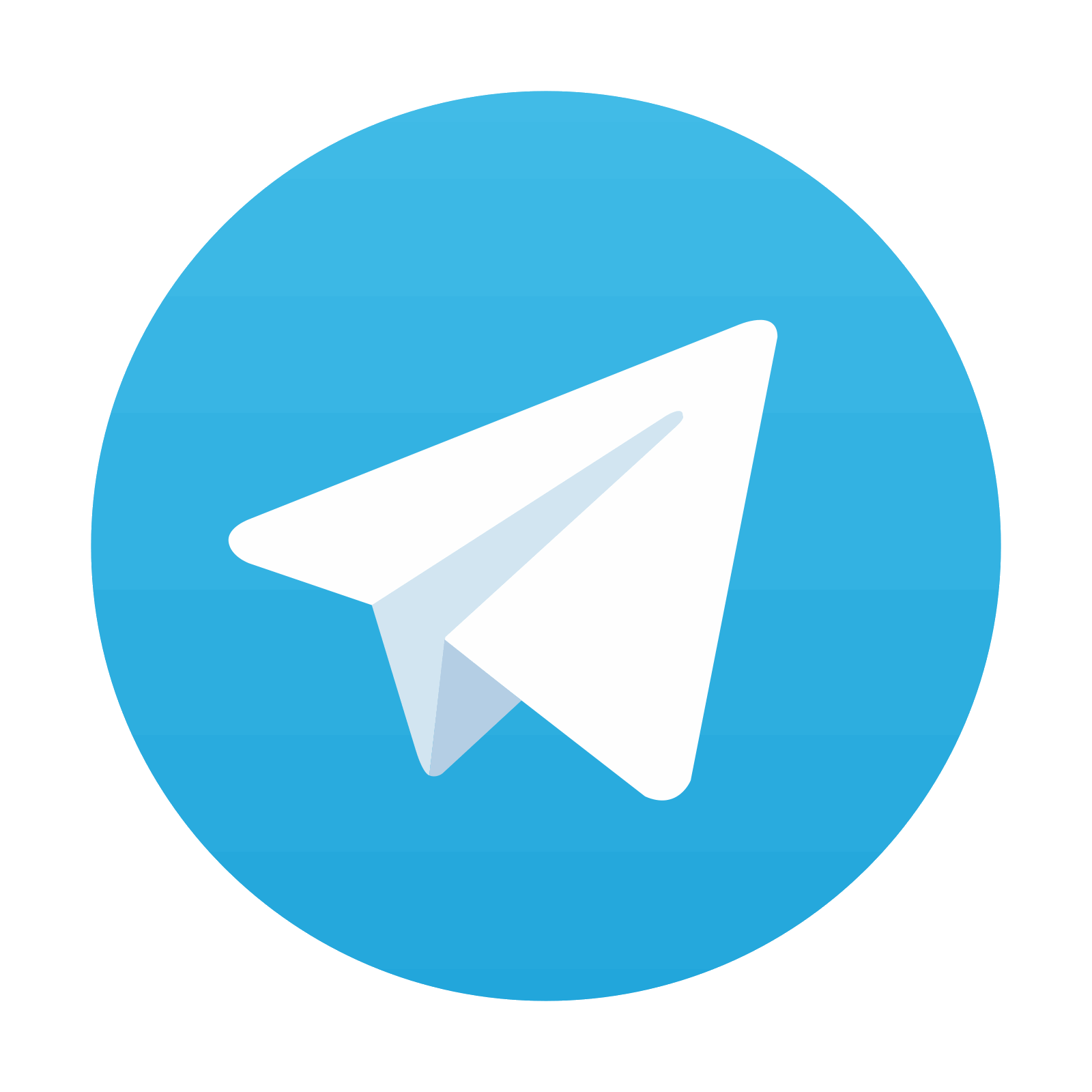
Stay updated, free articles. Join our Telegram channel
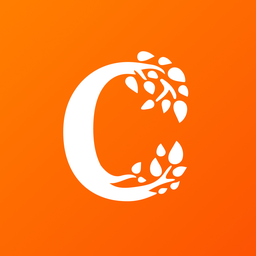
Full access? Get Clinical Tree
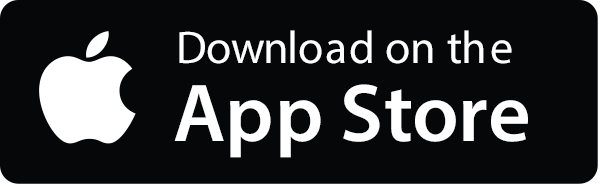
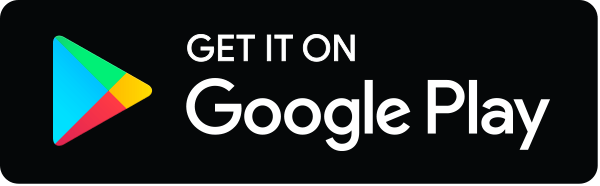