Introduction
The optic nerves carry the axons of retinal ganglion cells (RGCs), and these axons transmit all the visual information from the inner retina to the brain. The retina and optic nerve are developmentally an outgrowth of the forebrain, and, like other white matter tracts of the central nervous system (CNS), the optic nerve does not repair itself after most types of injury. Thus, diseases that affect the optic nerve commonly cause vision loss, and blindness from optic nerve injury or degenerative disease is typically irreversible. This chapter reviews the principal aspects of optic nerve anatomy, development, and physiology, and discusses the pathologic changes at the molecular and cellular levels in the context of clinical disease.
Optic nerve anatomy
Retinal ganglion cell axons within the nerve fiber layer
RGC axons begin at the RGC cell bodies in the innermost layer of the retina. Although most neurons in this layer are RGCs and the layer is commonly referred to as the ganglion cell layer , in humans approximately 3% of cells in the central retina and up to 80% in the peripheral retina may be other cell types, primarily “displaced” amacrine cells. In addition, studies in mammals have demonstrated the presence of “displaced” RGCs located in the inner nuclear layer. As discussed in Chapter 21 , each RGC receives input from bipolar cells and amacrine cells, and projects its axon toward the vitreous, whereupon the axon turns approximately 90 degrees and projects toward the optic nerve head in the nerve fiber layer. The nerve fiber layer is not quite radially arranged around the optic nerve head (optic disc), as axons temporal to the foveal center of the retina course away from the fovea, and then toward the optic disc, entering in the superior and inferior portions of the disc ( Fig. 28.1 ). This interesting anatomy prevents axons from crossing the high-sensitivity fovea, where they might otherwise scatter light and degrade visual acuity. The axons from more peripheral RGCs are more superficial (vitread) to those arising from less peripheral ganglion cells ( Fig. 28.2 ). In addition, there is strict segregation of those fibers arising from RGCs located superior to the temporal horizontal meridian (raphe) and those fibers arising from RGCs located inferior to the horizontal raphe. Because of this anatomical segregation of nerve fiber bundles, visual field defects corresponding to RGC axon injury typically have stereotyped patterns, for example, superior or inferior nasal steps, temporal wedges, or arcuate scotomas, that do not cross the horizontal meridian ( Box 28.1 ).


The number of retinal ganglion cell (RGC) axons in the optic nerve is, in most cases, probably well-approximated by the number of RGC axons in the nerve fiber layer of the retina immediately adjacent to the optic nerve. The peripapillary nerve fiber layer can be measured quantitatively using a variety of modalities including optical coherence tomography (OCT), which measures layer thickness based on reflectance of light from the interfaces between layers, and by scanning laser polarimetry (SLP), which estimates nerve fiber layer thickness based on the interaction of polarized light with organized bundles of microtubules inside axons. Each of these technologies may face limitations. OCT estimation of RGC axons may be complicated by the presence of retinal astrocytes, which accompany RGC axons through the nerve fiber layer and contribute to nerve fiber layer thickness. SLP estimation of axon integrity may be limited by degradation or disorganization of RGC microtubules that may precede axon loss. All such technologies are limited by their inability to measure optic nerve axons specifically, and rather substitute peripapillary axon measurements.
Intrascleral optic nerve
The optic nerve itself is considered to begin at the optic nerve head which, when viewed through the front of the eye, is observed as the optic disc. There is an approximately 1 mm component of optic nerve within the intrascleral part of the globe, which includes the lamina cribrosa ( Fig. 28.3 ). Once at the optic disc, ganglion cell axons turn away from the vitreous and dive into the optic disc toward the brain. Axons arising from more peripheral RGCs are peripheral within the optic nerve head. The optic disc contains the nerve fibers around its edge, the neuroretinal rim, and the central cup, which does not contain RGC axons. The cup-to-disc ratio may range from 0 to 1.0, depending on natural variation in the size of the disc, and whether there is less than a full complement of axon fibers, for example, as a result of damage from glaucomatous optic neuropathy (see further in chapter). More updated estimates of the complement of axon-containing tissue entering the intrascleral optic nerve include the Bruch’s membrane opening minimum rim width , typically measured from ocular coherence tomography (OCT) imaging.

Intraorbital optic nerve
The optic nerve extends approximately 30 mm from the globe to the optic canal. The straight-line distance from the back of the globe to the optic canal is much less (the exact amount depending on individual orbital depth), with the excess optic nerve laxity allowing for free movement of the globe during eye movements. In some cases excessive proptosis of the globe stretches the optic nerve tautly, resulting in direct injury to or even avulsion of the nerve itself. Beginning behind the globe, the nerve is ensheathed by the layered meninges extending from the brain, bathing the optic nerve in cerebrospinal fluid and providing vascular support along the length of the nerve (see further).
The initially retinotopic correspondence of optic nerve fibers to retinal location, including the segregation between axons arising from the superior and inferior retina, is gradually lost as the axons course through the nerve ( Fig. 28.2 ). There is only moderate retinotopy in the initial segment of the optic nerve. The retinotopy decreases distally, and then becomes ordered vertically for eventual nasal decussation near the chiasm. The loss of retinotopy is not absolute, because the fibers from the nasal (but not temporal) macula continue to be located centrally within the nerve over a considerable distance. Fibers from large RGCs are less retinotopically organized than those from smaller RGCs. Although studies in humans are difficult to do antemortem, postmortem studies of nerves with specific visual field defects demonstrate similar findings, and postmortem studies of developing fetuses and adult eyes also show loss of retinotopy within the transition from the optic nerve head to the more distal nerve. Within the orbit, the optic nerve travels within the muscle cone formed by the superior rectus, lateral rectus, inferior rectus, and medial rectus muscles. Tumors within the cone are common sources of compression of the optic nerve, or compressive optic neuropathy. Examples of these tumors include cavernous hemangioma, hemangiopericytoma, fibrous histiocytoma, lymphoma, and schwannoma. In addition, enlargement of the muscles themselves, particularly the inferior rectus and/or the medial rectus in Grave’s ophthalmopathy, may also compress the optic nerve.
The optic canal
The optic nerve enters the cranium via the optic canal, a 5- to 12-mm passage that lies immediately superonasal to the superior orbital fissure. The optic canal contains some axons of sympathetic neurons destined for the orbit, as well as the ophthalmic artery. The latter lies immediately inferolateral to the optic nerve itself, covered in dura. At the distal end of the canal, there is a half-moon–shaped segment of dura which overhangs the optic nerve superiorly, and thereby lengthens the canal by a few millimeters. As in the intraorbital portion of the optic nerve, within and immediately posterior to the optic canal, meningeal tissue ensheathes the optic nerve. Benign tumors of the meninges, or meningiomas, are frequent causes of compressive optic neuropathies in these locations. Small tumors within the canal itself, where there is very little free space, may lead to compressive optic neuropathy without a radiographically visible tumor.
Intracranial optic nerve and the optic chiasm
Once the nerve has entered the cranium, there is a highly variable length of nerve (8–19 mm, mean of 12 mm) until the optic chiasm is reached. The length of the chiasm itself is approximately 8 mm. The intracranial optic nerve and chiasm are immediately above the planum sphenoidale and sella turcica, the latter of which contains the pituitary gland. There is approximately 10 mm between the inferior part of the nerve and the superior part of the pituitary. Tumors of the pituitary that increase its size enough to impinge upon the chiasm cause compressive optic neuropathy.
At the optic chiasm, RGC axons from the temporal retina remain ipsilateral, and those from the nasal retina cross the chiasm and course toward the contralateral brain; in humans this ratio has been estimated at 47% ipsilateral anatomically and 48% ipsilateral functionally. This small difference between the number of crossing and noncrossing fibers is commonly reported to be responsible for the relative afferent pupillary defect seen in disorders of the optic tract, in which an afferent pupillary defect is seen contralateral to the injured tract, but may reflect the fact that some fibers from specialized cells within the retina responsible for the pupillary reflex may cross from the temporal retina into the contralateral optic tract.
The optic tract and lateral geniculate nucleus
Although the optic nerve ends anatomically at the chiasm, the RGC axons continue within the optic tract to the lateral geniculate nucleus (LGN), superior colliculus, pretectal nuclei, or hypothalamus (see further). Circuitry and processing by some of these targets are discussed in Chapters 27 and 29 .
Optic nerve axon counts and dimensions
In the normal adult human optic nerve, manual techniques have demonstrated an estimated 1,200,000 RGC axons per nerve. There is a strong correlation between the size of the neuroretinal rim and the number of axons, and between the number of axons and the size of the scleral canal in primates, although the degree of correlation is controversial.
Many factors affect the number of axons within the optic nerve, from inherited differences to damage from diseases, i.e., optic neuropathies (see further in chapter). In addition, there is a gradual loss of RGCs during normal human aging, with approximately 5000 to 7000 axons lost per year of life. For unclear reasons, there is a smaller degree of loss of macular RGCs with age compared with peripheral RGCs, and this may reflect contraction of the macula with time.
Although the number of RGC axons entering the optic nerve is fairly constant, the diameter of the optic nerve varies widely. At the disc itself, where the fibers are completely unmyelinated, the mean vertical diameter of the disc is 1.9 mm (range 1.0–3.0 mm) and the mean horizontal diameter is 1.7–1.8 mm (range 0.9–2.6 mm). The mean area of the disc is 2.7 mm 2 (range 0.8–5.5 mm 2 ). The mean area of the neuroretinal rim (not including the cup) is 2.0 mm 2 (range 0.8–4.7 mm 2 ). Because the axonal tissue entering the optic disc varies much less than the size of the optic disc itself, the optic cup in the center of the disc can vary greatly without necessarily reflecting any underlying deficit in the number of RGC axons. The diameter of the optic nerve approximately doubles posterior to the globe as a result of myelination of the axons.
Microscopic anatomy and cytology
Axons
There are no other neuronal cell bodies within the optic nerve, making it a pure white matter tract of the CNS. Although the optic nerve itself may contain other small nerves, particularly tiny peripheral nerves (branches of the trigeminal system) which carry pain sensation or control vascular tone, the vast majority of the optic nerve is composed of the approximately 1,200,000 axons of the RGCs. Optic nerve axons are collected in fascicles, which are separated by pia-derived septa. The number of fascicles ranges from approximately 50 to 300, maximal immediately retrobulbar and at the optic canal. The mean axon diameter is slightly less than 1 µm, with a unimodal skewed distribution. Compared with the optic tract, there is relatively little segregation of axons by size within the optic nerve, except for a tendency for thinner axons to be located inferocentrotemporally.
There is variability of axonal diameter and myelin thickness from the retina to the brain. Studies in other mammals show that the diameters of the largest axons increase as the distance from the retina increases. The diameter of individual axons is regulated by multiple factors including oligodendrocytes and electrical activity, increases during development, and decreases during aging, either through loss of large-diameter axons or from a general, aging-induced atrophy.
Oligodendrocytes and myelin
Conduction of nerve impulses down the axons in the optic nerve depends on the presence of myelin, a fatty, multilaminated structure which insulates each axon and greatly increases the speed and efficiency of conduction (discussed further). The RGC axons in the retrolaminar optic nerve are completely myelinated under nonpathologic circumstances ( Fig. 28.4 ). The occasional axons of the peripheral nervous system running within the adventitia of the central retinal artery and in the outer dura may be nonmyelinated or myelinated by peripheral nerve Schwann cells. Each axon is myelinated with several lamellae of myelin bilayers, the number of which vary from axon to axon but in proportion to the diameter of the axon. Individual oligodendrocytes elaborate an average of 20 to 30 processes per oligodendrocyte, and each process myelinates 150 to 200 microns of axon length.

Oligodendrocytes and axons regulate each other during development and throughout adulthood. Oligodendrocytes depend on the presence of axons for their survival and production of myelin proteins. Axons regulate oligodendrocyte survival and proliferation, and thus their number, through expression of specific signaling proteins and through axonal electrical activity, thus controlling oligodendrocyte numbers to match the number of axons. Conversely, axons are signaled by oligodendrocytes to regulate axon diameter, and to prevent axons sprouting or branching within the optic nerve. Later, in the adult, oligodendrocytes and myelin are partially responsible for inhibition of axon regeneration after optic nerve damage or degeneration (see further).
Astrocytes
Astrocytes, named for their stellate appearance, are ubiquitous glial components of the CNS and function in white matter tracts such as the optic nerve to regulate ionic and energy homeostasis. Astrocytes are highly efficient at transporting potassium, and increases in the level of extracellular potassium as a result of axon repolarization are buffered by astrocytes. Their ability to accumulate glycogen may allow them to serve as an energy source for the optic nerve in the absence of glucose (e.g., ischemia), by shuttling lactate to adjacent axons, a mechanism that may play a role in injury response. Astrocytes form the glial-limiting membrane, and their processes are concentrated at the nodes of Ranvier and in contact with nearby capillaries. This positions the astrocyte to play a role in transportation of substances between the local circulation and the axons; in inducing endothelial cells to form the blood-brain barrier ; and perhaps in signaling blood vessels to dilate or constrict according to local metabolic needs. Astrocytes also mediate connectivity between optic nerve axons and the adjacent connective tissues, such as the pial septa, the adventitia of the central retinal artery and vein, and the pia in a layer named the glial mantle of Fuchs. Recent data in rodents suggest that optic nerve head astrocytes also play a role in phagocytosing discarded material from axons, including extruded degenerating mitochondria.
Interestingly, the most common intrinsic tumor of the optic nerve is astrocytoma, or optic nerve glioma. This is usually a low-grade tumor of well-differentiated astrocytic cells that appear hair-like, or “pilocytic.” Pilocytic astrocytomas are usually seen in childhood and have a favorable prognosis. They are also commonly seen in association with neurofibromatosis. Rarely, more malignant neoplasms of astrocytic origin develop in adults. These resemble the higher-grade astrocytic neoplasms found elsewhere in the CNS, and are usually fatal.
Microglia
Microglia, a type of resident macrophage, are an important cellular component of the optic nerve. Although their origin was debated for decades, they have been shown experimentally to be of peripheral, bone marrow origin, and are not derived from the neuroectoderm that yields neurons, astrocytes, and oligodendrocytes. Microglia share several markers with macrophages, with both having Fc receptors (for immunoglobulin), C3 receptors (for complement), binding of Griffonia isolectin B4, and antigenicity for F4/80 and ED1 monoclonal antibodies. In the human optic nerve, microglia can be seen at 8 weeks after conception, when they are relatively undifferentiated. Microglia become more differentiated during fetal development, going from tuberous to amoeboid to a ramified morphology. They are associated with axon bundles, but not necessarily with blood vessels, and are found in both the nerve parenchyma and its meninges. Nerve and meningeal microglia are similar ultrastructurally except for vacuoles and endoplasmic reticulum in the former. This may be due to their phagocytosis of dying axons during development. Microglia share several characteristics of immune capacities of macrophages. By phagocytosing extracellular material, degradation within intracellular compartments can occur. This may be followed by antigen presentation on the cell surface. In combination with certain histocompatibility antigens, this presented antigen can cause stimulation of T lymphocytes and subsequent immune system activation (see further).
Meninges and meningothelial cells
The optic nerve is covered with three layers of meninges: dura, arachnoid, and pia. The meninges can also be divided up into pachymeninges (dura) and leptomeninges (arachnoid and pia). The outermost dura is a thick fibrovascular tissue, which is in immediate contiguity with the sclera, the periorbita, and the dural layer of the lining of the cranial contents. The middle arachnoid layer is a loose, thin, fibrovascular tissue. The innermost pia is a thin, tightly adherent layer with extensions into the nerve itself forming the pial septae, through which the fascicles of ganglion cell axons course. In the optic canal, there are numerous trabeculae connecting the dura through the arachnoid to the pia, which reduce the free space of the nerve sheath in this area.
The space between the dura and arachnoid is the subdural space, while the space between the arachnoid and pia is the subarachnoid space. The subdural space around the optic nerve is small, and is not in communication with the intracranial subdural space. Meningothelial cells may have several functions including that of wound repair and scarring, phagocytosis, and collagen production. The subarachnoid space, on the other hand, is in communication with the intracranial subarachnoid space. The optic nerve subarachnoid space ends anteriorly within a blind pouch just before the optic disc. There is an appreciable pressure within the subarachnoid space, measuring from 4 to 14 mmHg. This extension of the subarachnoid space from the brain into the orbit explains why elevated intracranial pressure may cause compression of the optic nerve by elevating the hydraulic pressure, and differences between intracranial pressure and intraocular pressure (IOP), measured across the thin tissue of the optic nerve head, are now linked to the pathophysiology of glaucoma.
Blood supply
Optic nerve head
Studies of histologic sections and of corrosion casting of the vessels themselves have added greatly to our understanding of the optic nerve blood supply ( Fig. 28.5 ). The ophthalmic artery provides the major vascular supply to the inner retina and optic nerve. The central retinal artery branches off from the intraorbital ophthalmic artery and enters the optic nerve approximately 12 mm behind the globe. In the retina, RGC bodies and the nerve fiber layer are supplied by capillary branches derived primarily from the central retinal artery arising out of the optic nerve head. As it branches on the optic disc into the retinal arterioles, the central retinal artery provides partial perfusion of the superficial optic disc via small capillaries. Along the optic nerve itself, however, the central retinal artery provides minimal perfusion of the nerve.

In contrast, branches of the medial and lateral short posterior ciliary arteries, originating from the ophthalmic artery, as well as the choroid, provide the major blood supply to the optic nerve head. Their branches perfuse the optic nerve, both anteriorly via direct branches and posteriorly via a retrograde arteriolar investiture of the optic nerve (see further). Anastomoses of posterior ciliary artery branches form the circle of Zinn-Haller, which contributes significant perfusion to the optic nerve head. In addition, there are contributions from recurrent choroidal arterioles to the prelaminar and laminar optic nerve head, and contributions from recurrent pial arterioles to the laminar and retrolaminar optic nerve head. A clinical implication of the common posterior ciliary arterial source of the choroid and deep optic nerve head is that they will fluoresce simultaneously during the earliest phase of fluorescein angiography, before the retinal arterioles transit dye.
Unlike the choroidal vessels, which have fenestrated endothelial cells, optic nerve vessels have nonfenestrated endothelial cells with tight junctions, surrounded by pericytes. Optic nerve vessels therefore share the same blood-nerve barrier characteristics as the blood-brain barrier. Only a restricted number of molecules can cross the blood-nerve barrier. For example, gadolinium enhancement on magnetic resonance imaging is not seen unless there is some pathologic process within the optic nerve that would disrupt the blood-nerve barrier, for example, inflammation. Another major difference between choroidal and optic nerve head vessels is that only the latter can autoregulate, i.e., maintain approximately constant blood flow despite most changes in intravascular or extravascular (intraocular) pressure. Thus there is compensation of perfusion for a wide range of IOPs in normal individuals. A dysregulation in optic nerve head autoregulation, and any ischemia that would follow, may contribute to the pathophysiology of glaucoma (see further).
Intraorbital optic nerve and optic canal
The intraorbital optic nerve is perfused primarily by the pial circulation, which branches from the ophthalmic artery either directly or indirectly via recurrent branches of the short posterior ciliary arteries. The pia sends penetrating vessels into the intraorbital optic nerve along the fibrovascular pial septae, from which a capillary network extends into neural tissue (axons and glia). Similarly, the intracanalicular optic nerve is perfused by up to three branches of the ophthalmic artery, namely a medial collateral, lateral collateral, and ventral branch, which perfuse the pial surface and then penetrate the nerve. An important clinical implication of the pial supply relates to optic nerve sheath meningiomas. If a surgeon strips the meningioma away from the nerve, then the pia will also be removed, resulting in loss of the blood supply to the nerve and possible infarction and blindness. Another clinical correlation is related to the small amount of free space within the canal, which when occupied by hematoma from shearing of the delicate vessels there may result in a sight-threatening compressive hematoma (and see further).
Intracranial optic nerve, chiasm, and optic tract
The intracranial optic nerve and chiasm are perfused by the internal carotid artery and its branches, primarily the anterior cerebral, the anterior communicating, and the superior hypophyseal artery. The posterior chiasm may also be perfused by branches of the posterior communicating artery. The optic tract is predominantly perfused by branches of the posterior communicating and anterior choroidal arteries. Similar to the intraorbital and intracanalicular optic nerve, the blood supply occurs via small pial penetrating vessels. However, there is no dura or arachnoid surrounding the optic nerve posterior to the optic canal, and thus the risk of infarction is not from stripping a tumor off the nerve, but from inadvertent detachment of the fine vessels during surgical manipulation.
Vascular biology
Vessels of the human optic nerve contain endothelial cells separated from pericytes by a basement membrane, with endfeet of astrocytes surrounding the two. The tight junctions between endothelial cells contribute to the blood-nerve barrier, which is present in the normal optic nerve throughout its length. The anterior part of the optic nerve, however, shares a distinctive feature with the area postrema, choroid plexus, and median eminence, in that there is local disturbance of the blood-brain barrier. Although the optic nerve capillary endothelial cells in the anterior optic nerve have tight junctions, leakage can occur from adjacent choriocapillaris through the border tissue of Elschnig at the level of the lamina choroidalis. Further leakage from this area into the subretinal space may be prevented by tight junctions between the glia making up the intermediary tissue of Kuhnt. The blood-nerve barrier may be incompetent in focal inflammatory disorders such as optic neuritis.
The optic nerve differs from almost all other areas of the CNS in that there is connective tissue around vessels. The vessels are closely surrounded by morphologically specialized collagen and pial fibroblasts, beyond which are astrocytic foot processes. These pial septa are not a constant factor of mammalian optic nerves; rats, for example, do not have septa.
The vessels within the nerve have their own innervation. These nervi vasorum contain multiple neurotransmitters including adrenergic, cholinergic, and calcitonin gene–related peptide; neuropeptide Y; substance P; and vasoactive intestinal peptide. Human posterior ciliary arteries respond to the potent vasoconstrictor angiotensin II.
Optic nerve development
Generation of optic nerve oligodendrocytes and myelination
Oligodendrocytes develop from oligodendrocyte precursor cells (OPCs). OPCs migrate from the brain into the optic nerve, perhaps from the base of the preoptic recess, and this migration may continue during adult life. The OPC has a complex regulatory mechanism, with in vitro and animal studies implicating platelet-derived growth factor (PDGF) and ciliary neurotrophic factor (CNTF). Other factors in the differential control of differentiation, division, and renewal of the stem cell population, such as basic fibroblast growth factor, are likewise involved. An intrinsic developmental clock controls the differentiation of OPCs into oligodendrocytes, after which the cell is mitotically unresponsive to PDGF. In normal development, some OPCs persist into the adult and may participate in remyelination.
After oligodendrocyte differentiation from OPCs, there is typically a short delay before myelination starts, which may be regulated by inhibitory signals from axons activating the notch signaling pathway. The developmental regulation of myelination of the optic nerve and tract occurs earliest at the brain end of the optic nerve, then travels distally toward the eye, (i.e., proximally with respect to the ganglion cell axon). This is in contradistinction to myelination elsewhere in the CNS, which usually occurs from the neuron cell body outward along the axon. The brain-to-eye direction of myelination correlates with animal studies demonstrating OPC migration and then differentiation outward along the optic nerve. In humans, oligodendrocytes are not seen in the optic nerve before 18 weeks of gestation. Later, optic tract and intracranial nerve axons begin myelination at 32 weeks of gestation, with the process virtually complete at birth. The axons adjacent to the globe begin myelination only at birth; all are myelinated by about 7 months of age. Further myelination continues, however, and thickening of axonal myelin may continue past 2 years or longer.
In longitudinal studies of rat optic nerve development, axons that become myelinated increase in diameter. The signal for change in caliber is generated by the oligodendrocyte. Although unmyelinated and not yet myelinated axons can conduct electrical impulses in the developing optic nerve, there is a surprising increase in conduction velocity during development, independent of diameter or myelination status, the reasons for which are unclear.
Interestingly, intraretinal RGC axons are not normally myelinated in most species; indeed, there are normally no oligodendrocytes in the retina. Studies of rat optic nerve head and anterior optic nerve demonstrate an abnormal transition zone from unmyelinated axons to myelinated axons, with varying lengths and thicknesses of myelin, unusual nodal region morphology, and few normal oligodendrocytes. Studies of rat optic nerve suggest that a signal found at the lamina cribrosa blocks the migration of OPCs into the retina. This barrier is possibly due to the presence of specialized optic nerve head astrocytes, serum factors from a focally deficient blood-nerve barrier or the adhesion substrate tenascin. In rabbits, which have no lamina cribrosa, there are axons myelinated by oligodendrocytes in the retina, and intraretinal OPCs can be identified. Further, the retinal portion of the ganglion cell axon has no constitutive barrier to myelination because ganglion cell axons of retina transplanted into rat midbrain can be myelinated, and OPCs injected intraretinally can differentiate and myelinate RGC axons. Myelination may also be artifactually induced by injuring the retina from the scleral side. This trauma leads to migration of Schwann cells and subsequent intraretinal myelination. In human eyes, myelination of part of the nerve fiber layer is occasionally seen; it is assumed that this is secondary to ectopic oligodendrocytes, although there are reports of Schwann cells myelinating intraretinal axons in the cat and rat. Although oligodendrogliomas within other parts of the CNS are sometimes seen, neoplasms of oligodendrocytes within the optic nerve are very rare.
Generation of optic nerve astrocytes
Astrocyte precursor cells have been considerably less well studied. Like oligodendrocytes, developmental regulation of astrocyte number is determined by RGCs. Astrocyte precursor cells may depend on CNTF or leukemia inhibitory factor (LIF) for differentiation into astrocytes, at which point they upregulate the expression of glial fibrillary acidic protein (GFAP), an intermediate filament protein. GFAP itself is important to optic nerve development, as transgenic mice lacking GFAP demonstrate abnormal myelination and blood-brain barriers. Astrocytes may also be critical for optic nerve development in that they may serve as the substrate over which extending ganglion cell axons grow.
Development of optic nerve meninges
The anatomy and development of the optic nerve meninges have been well described. All three meningeal layers are formed from fibroblast-like cells, which vary in ultrastructural appearance from typical process-bearing fibroblasts to mesothelial cells lining the subdural and subarachnoid space. The meningeal layers are particularly rich in collagen and elastin. Trabeculae between the arachnoid and pia are formed from collagen and are lined with the same mesothelial cells that line the subarachnoid space. The most rapid proliferation of human meningeal cells during development occurs within 8 to 18 weeks of conception, with centrally located fibroblasts and peripherally located cells containing glycogen that increase during this time. By 14 weeks, the three layers of the meninges can be distinguished. These glycogen-rich cells go on to produce large amounts of collagen, which is deposited in the dura after 14 or 15 weeks.
Axon number
Studies in animals suggest that approximately 50% to 100% excess RGCs are produced in the retina. During early development, the number of RGCs decreases by programmed cell death called apoptosis (discussed in the context of axon injury, further), in many cases because not all of the axons properly connect to their target regions within the brain. This form of programmed cell death may be adaptive if it does not release toxic intracellular components into the extracellular environment and thereby does not affect neighboring cells that are already integrated to survive. Likewise, in humans, up to two-thirds of developing RGCs die, predominantly during the second trimester.
Axon growth
Considerable progress has been made in recent years in our understanding of the development of the optic nerve, particularly at the level of molecular and cellular regulation. Axon growth requires specific extracellular signals—merely inhibiting cell death is not sufficient for inducing axon growth in developing RGCs. The most potent signals for axon growth are the same peptide trophic factors that most strongly promote RGC survival, and multiple trophic factors can often combine to induce even greater axon growth. The best studied peptide trophic factors for RGC axon growth are brain-derived neurotrophic factor (BDNF), CNTF, insulin-like growth factor (IGF), basic fibroblast growth factor (bFGF), and glial cell line–derived neurotrophic factor (GDNF), although it is not clear how critical any of these are singly for axon growth during normal development. Other than peptide trophic factors, extracellular matrix molecules such as laminin and heparin sulfate proteoglycans, and cell adhesion molecules such as L1 and N-cadherin are expressed in the visual pathway and provide a critical substrate along which axons extend.
Electrical activity (discussed further in the chapter) is not required for axon growth, but likely plays a role in sculpting axonal morphology. After injecting tetrodotoxin into the eye to block action potentials, or in experimental mice in which synaptic release of neurotransmitters is essentially eliminated, RGCs and other CNS neurons successfully elongate their axons to their targets in the absence of electrical activity. RGC activity may increase the rate of axon arborization in their target fields by stabilizing growing branches, and may influence the specificity of local connectivity and targeting of axons. Considerable progress has been made in understanding these molecular mechanisms for axon growth, and these have been reviewed elsewhere.
Axon guidance
Optic nerve axon guidance is heavily influenced by the earliest pathfinding axons, which serve as a substrate and guidance cue for the growth of the axons that follow. During development, RGC axons are guided by neuronal and glial molecules that attract and repel axonal growth cones. For example, embryonic RGC axons are repelled by Müller glia away from deeper retinal layers into the nerve fiber layer. Within the retina, the interesting course of retinal axons in the macular nerve fiber layer is likely due to a foveal repellant combined with an optic disc attractant. RGC axons are directed centrally in the retina away from chondroitin sulfate proteoglycans (CSPGs) and are attracted into the optic nerve head by netrins. A semaphorin, sema5A, may contribute to keeping the developing axons within the substance of the developing optic nerve. Ipsilateral-projecting axons are repelled at the optic chiasm by Slit1 and Slit2, ephrin-B, and CSPGs. The developmental ordering of the axons as they pass through the chiasm and go on to innervate the LGN primarily reflects their time of arrival.
RGC axons reach four main targets: (1) the LGN of the thalamus; (2) the superior colliculus; (3) the pretectal nucleus; and (4) the suprachiasmatic nucleus, although several other targets exist. Most axons course unbranched to the LGN, but studies in many animals, including primates, have demonstrated the existence of axon collaterals to the other targets, suggesting that the same may be true for human optic nerves.
The LGN is the primary synaptic target for visual information processing on the way to the visual cortex (discussed at length in Chapter 29 ). The primate LGN has six layers: layers 1 and 2 consist of large (magnocellular) cells, and layers 3, 4, 5, and 6 consist of small (parvocellular) cells. The magnocellular layers receive input from the midget RGCs, while the parvocellular layers receive input from the parasol RGCs. Layers 2, 3, and 5 receive input from the ipsilateral retina, while layers 1, 4, and 6 receive input from the contralateral retina. In addition, there are interlaminar cells forming the koniocellular layers. These cells are smaller than those of the parvocellular layers, and receive synaptic input in part from blue-yellow RGCs.
A second set of optic nerve axons project to the superior colliculus, a paired structure of the dorsal midbrain. In lower animals, for example, rodents, the majority of fibers of the optic nerve project to the superior colliculus. In primates, including humans, only a minority does so, with the majority of axons destined instead for the LGN. The superior colliculus has a laminar organization and is retinotopically mapped. Most studies of superior colliculus architecture have been done in animals, although the human superior colliculus appears to have similar anatomy. The function of the superior colliculus is to coordinate retinal and cortical control of saccades and fixation. Fibers branching to midbrain terminal nuclei stabilize the retinal image during movement of the eye, head, or body.
The pretectal nucleus in the midbrain receives RGC fibers important for the pupillary response. The pretectal projection is bilateral, and in addition, fibers from each pretectal nucleus project both ipsilaterally and contralaterally to the Edinger-Westphal subnuclei of the third nerve nucleus. The latter then projects parasympathetic axons along the course of the third nerve to the ciliary ganglion within the orbit, where a synapse is made. Postsynaptic parasympathetic fibers continue to the pupilloconstrictor muscle, constriction of which is visible as the pupillary light reflex. The combined ipsilateral and contralateral projections from the retina to the pretectal nuclei, and from the pretectal nuclei to the Edinger-Westphal subnuclei necessitate that a light stimulus causing impulses along either optic nerve will cause equal constriction of both pupils. This is the physiologic basis for detecting an afferent pupillary defect (also called the swinging flashlight test or Marcus Gunn pupil). If there is a functional difference in the number of optic nerve axons carrying impulses from each retina, then light shined into the eye with fewer axons will cause a lesser amount of (bilaterally symmetric) pupillary constriction than light shined into the other eye. By alternating the light between the two eyes and observing the difference in pupil size dependent on which eye the light is shined into, one can detect which optic nerve is functionally conducting less than the other. This measure is highly correlated with differences in the number of surviving RGCs. It has been proposed that there are differences between the myelin surrounding axons destined for the LGN and those responsible for the pupillomotor reflex because of the existence, albeit rare, of patients with demyelinative optic neuropathies and monocular blindness with preserved pupillary reflexes. More details about the pupil light reflex can be found in Chapter 25 .
A fourth projection of optic nerve axons is via the retinohypothalamic tract to the suprachiasmatic nucleus and the paraventricular nucleus within the hypothalamus. These fibers are responsible for circadian control of the sleep-wake cycle (mediated by a population of intrinsically photosensitive RGCs [ipRGCs] that express the photopigment melanopsin ), temperature, and other systemic functions. Roles for ipRGCs are described in detail in Chapter 26 .
Optic nerve physiology
Retinal ganglion cell electrophysiology and synaptic transmission
In the retina, RGCs receive synaptic input from bipolar and amacrine cells, which primarily use glutamate as the major excitatory neurotransmitter. RGCs have both NMDA and non-NMDA ionotropic glutamate receptors, as well as metabotropic receptors. Other neurotransmitters modulating RGCs include GABA, acetylcholine, and aspartate (acting on NMDA receptors). Within the retina itself the levels of glutamate are controlled by Müller cells, which have glutamate transporters, and which contain the enzyme glutamine synthetase, converting glutamate to the amino acid glutamine. Glutamate reuptake within the retina is necessary for maintaining appropriate RGC function. The electrophysiology of RGCs is complex, and is discussed at length in Chapter 23 and elsewhere. RGCs themselves are glutamatergic, but they also use other neurotransmitters, including substance P and serotonin. Furthermore, RGCs are extensively interconnected with other RGCs and amacrine cells via gap junctions, which enables sharing of electrical signals but also affects susceptibility to trauma.
Axonal conduction
Action potentials
RGC axons transmit information via action potentials, which are all-or-nothing spikes of electrical activity. This is in contrast with intercellular communication within the retina, where graded potentials are predominantly used to transmit information. With action potentials, the actual amount of voltage change, (i.e., depolarization), is the same, while the number of impulses per second and the distribution of impulses within various axons is the mechanism by which visual information is carried down the optic nerve. Conduction down individual axons occurs via the same biophysical mechanisms which occur in any myelinated axon (see section on myelin further in the chapter). Variations in conduction velocity of individual axons may help coordinate conduction time between RGCs located at differing distances between their retinal location and the optic nerve.
The mechanism of axonal conduction is straightforward. At rest, the inside of an axon is at a negative voltage with respect to the outside of the axon. This resting potential is negative, and primarily results from the fact that the concentration of potassium is much higher within the axon compared with its extracellular concentration. As a small number of potassium ions flow down their concentration gradient from the inside of the axon to the outside, this movement of positive charge out of the axon results in a negative potential within the axon. Outward flux of potassium continues until the charge separation becomes too great and can no longer be driven by the concentration difference between the inside and outside of the axon. The point at which the gradient for potassium concentration is balanced by the gradient for separation of charge results in an equilibrium potential for potassium. While the resting potential is primarily determined by potassium, there is also a smaller contribution from sodium flowing down its concentration gradient. In the case of sodium, the concentration in the extracellular space is high, compared with a low concentration within the axon. This concentration gradient would induce sodium to enter the axon, and movement of a small number of sodium ions results in a positive sodium equilibrium potential. However, in a resting (nondepolarizing) axon the conductance for sodium is much smaller than that for potassium, and therefore the final resting potential is weighted much more by the equilibrium potential for potassium than for sodium.
The situation changes during axonal conduction. Partial axon depolarization (resulting from an upstream section of membrane that is already depolarized—with the initial trigger starting at the axon hillock near the cell body) induces opening of voltage-sensitive sodium channels located within the membrane. These allow much greater amounts of sodium to enter the axon, and the positive sodium ions cause the axon to become more positive, (i.e., depolarized). In this case, the potential across the membrane is now weighted far more by the sodium equilibrium potential than by the potassium equilibrium potential. Increased potential within the axon rapidly affects adjacent sections of the axon and causes sodium channels in downstream areas of the axonal membrane to likewise become partially depolarized, in turn initiating the process of activating voltage-gated sodium channels. This chain of events continues down the axon, resulting in long-range transmission of an action potential.
Repolarization is the return of the axonal membrane potential to the original (negative resting potential) state and is necessary for more than a single action potential to be transmitted down the axon (while also ensuring action potentials do not propagate in the incorrect direction). Repolarization is due to the closure of the voltage-sensitive sodium channels and a transient opening of voltage-sensitive potassium channels. Once the latter occurs, the membrane resting potential is weighted more by the potassium equilibrium potential than by the sodium equilibrium potential. This results in a restoration of membrane potential to the resting state.
In the process of transmission of an action potential there is movement of sodium and potassium ions along their concentration gradients. If action potentials continued indefinitely, the sodium and potassium concentrations would reach equilibrium across the axonal membrane, resulting in loss of their concentration gradients and blocking conduction. Therefore, in order to re-establish their concentration gradients, there is a relatively slow redistribution process of these ions via the Na + K + -ATPase that takes place. This is a highly energy-dependent process and will fail to happen if axonal metabolism is significantly disturbed.
Role of oligodendrocytes and myelin
Myelin confers two electrophysiological functions. First, it decreases capacitance, meaning less sodium ion positive charge needs to enter the axon to depolarize the membrane. Second, it increases resistance, meaning that there is less leakage of charge across the membrane. Together, these properties decrease the amount of ionic flux needed to achieve changes in voltage across the membrane, saving on Na + K + -ATPase activity and thus energy needed to maintain ionic homeostasis after conduction.
Oligodendrocytes and myelin confer other critical properties for axonal conduction. Neonatal unmyelinated axons have a low density of sodium channels spread along the length of the axon, which allows a measure of axon conduction. In adults, however, ion channels are not distributed uniformly along a myelinated axon. Instead, the channels are segregated into patches located within the small areas where the axon is unmyelinated, called nodes of Ranvier. Conduction in a myelinated axon, called saltatory conduction, becomes much faster, as depolarization “jumps” from one node to the next. The clustering of sodium channels into nodes, as well as an important developmental switch in sodium channel isoforms, are also induced by oligodendrocytes.
Role of astrocytes
Although neurons are classically considered the only electrically active cell population in the CNS, astrocytes also have ion channels. For example, multiple voltage-sensitive sodium channels have been demonstrated in rat optic nerve astrocytes. Both sodium and potassium channels have been studied electrophysiologically in cultured astrocytes. Within the intact optic nerve, nonvesicularly released glutamate from the axon may induce glial cell spiking and possibly other effects. Whether ion channels or neurotransmitter receptors on optic nerve glia are physiologically important in vivo has yet to be demonstrated, but their presence suggests that optic nerve astrocytes may be more functionally specialized than previously recognized.
Axonal transport
The entire length of the RGC axon must be maintained by transporting proteins, mRNA, mitochondria, and other subcellular constituents many centimeters from the cell body, where the nucleus and most of the protein synthetic machinery resides. Axonal transport occurs in two directions, orthograde (away from the cell body and toward the brain), and retrograde (toward the cell body and away from the brain). By injecting radioactive, fluorescent, or enzymatically active macromolecular tracers into either the eye or the terminal fields of the axons, the course and timing of orthograde and retrograde transport can be determined. The rates of transport of specific moieties may vary as a function of stage of optic nerve development, suggesting a developmental regulation of axonal transport. Different rates of axonal transport may be related to differences in the motor proteins interacting with microtubules, for example, kinesin for fast transport and dynein for slow transport.
Fast axonal transport, at 90 to 350 mm/day, carries several subcellular organelles, such as the vesicles of neurotransmitter used in synaptic transmission, toward the axon terminal. This class of transport continues despite transection of the axon distal to the cell body, suggesting that intra-axonal components are sufficient for the process to occur. Slow axonal transport is divided into two classes, a slower class at 0.2 to 1 mm/day that carries cytoskeletal proteins, such as neurofilament proteins and the tubulins that make up microtubules, and a more rapid class at 2 to 8 mm/day, which carries proteins such as actin and myosin, metabolic enzymes, and mitochondria.
Retrograde transport occurs at about half the velocity of fast orthograde transport. Retrograde transport of neurotrophic molecules during development may signal the cell body that the axon is reaching its targets in the brain. How do growth signals at the axon get passed along to the distant cell body, where gene transcription and translation are induced to support survival and axon growth? Neurotrophin-mediated activation of Trk receptors leads to endocytosis of activated, ligand-bound receptors into clathrin-coated vesicles. These signaling endosomes carry with them the machinery of the ras-raf-MAP kinase signaling cascades, and can continue to activate these pathways once inside the cell. Neurotrophin signaling endosomes may then be retrogradely transported back to the cell body along microtubules, where they can activate transcriptional regulators and induce new gene expression.
Recent studies in rodent optic nerve have greatly expanded the characterization and understanding of the mRNAs and proteins transported down axons. With advances in mass spectrometry and RNA sequencing, the cohort of transported proteins has been named the axon transportome . Differential transport of mitochondria, proteins of various classes, and mRNAs during axon development, in response to visual/electrical activity, and after injury explain changing physiology of the axon and likely the axon terminals and synapses in various brain regions.
Optic nerve injury
Clinical implications
The optic nerve is commonly involved in disease, resulting in optic neuropathy and vision loss. Glaucoma is the most common optic neuropathy; others include inflammatory optic neuropathies such as optic neuritis associated with the demyelinating disease multiple sclerosis; ischemic optic neuropathy usually affecting older adults; and compressive optic neuropathy, commonly associated with a tumor or aneurysm. Most optic neuropathies are due to damage to the optic nerve, or pathophysiology occurring at least partially in the optic nerve.
Types of optic nerve injury
Traumatic optic neuropathy
Although traumatic optic neuropathy, particularly optic nerve transection, is one of the least common human scenarios in which RGC axons are injured, it has been the best studied animal model by far. In humans, concussive injury can indirectly traumatize the optic nerve and injure axons, as might happen after a car accident or other blunt injury. Direct transection, although rarer, might result from a foreign body such as a bullet or a fragment of broken orbital bone, or in optic nerve avulsion. In animal models, typically the optic nerve is surgically cut or crushed within the orbit behind the eye, although approaches crushing the nerve intracranially or traumatizing the optic nerve using concussive injury have also been studied. Regenerative response is assayed by labeling RGC axons and determining whether any extend past the lesion site. Interestingly, although injuring the optic nerve at increasing distances from the eye delays the onset of RGC death, there may be less regenerative response with more distal injury.
Ischemic optic neuropathy
Because the vascular supply to the optic nerve is complex and varies qualitatively along its course, a variety of clinical syndromes may result from ischemia or infarction at each location. Arteritic and nonarteritic anterior ischemic optic neuropathies are associated with histopathologically verifiable occlusion of posterior ciliary arteries. An infarction of the central retinal artery or one of its branches will result in RGC axon loss, with a pale disc and loss of axons within the optic nerve itself. Ischemia may also result from hypotension or severe blood loss, although the resulting pathophysiology may vary. Finally, there is controversy as to the degree to which the effects of IOP causing axonal damage in glaucomatous optic neuropathy are an ischemic or compressive process.
The pathophysiology of myelinated axonal ischemia is complex. The presence of myelination affects the metabolism of the axon. Because less current is necessary for depolarization to occur, less ATP is needed for transmission of action potentials. At the same time, the myelinated axon is more sensitive to anoxic damage. Although neonatal optic nerve, which is not myelinated, does not easily suffer irreversible damage from anoxia, more mature nerves from animals that have just undergone myelination are highly sensitive to anoxia. Similarly, optic nerves from md rats, a myelin-deficient rat strain, are sensitive to anoxia. Nonetheless, even myelinated axons (white matter) are relatively less sensitive to ischemia than neuronal cell bodies (gray matter).
Ischemic optic nerve injury can be modeled in experimental animals via a variety of approaches. A powerful method is to occlude the posterior ciliary arteries, best done in primate animals, but which has the disadvantage of also affecting the choroidal circulation. Infusion of the vasoconstrictor endothelin-1 into the perineural space is a good model of subacute to chronic ischemia, and can be performed in a variety of species. The best models involve direct vascular damage, tissue edema, and vascular compromise at the optic disc and at the retrobulbar optic nerve.
Optic neuritis and inflammation
Inflammation of the optic nerve, or optic neuritis, is the most common form of acute optic neuropathy in young and middle-aged adults, and is a frequent harbinger of multiple sclerosis. Demyelination is the most common pathologic accompaniment of optic nerve inflammation, and conduction block owing to this or other effects of inflammation is responsible for the usually temporary loss of visual function seen in patients with optic neuritis. Demyelination itself would not necessarily cause loss of RGCs, and, as in multiple sclerosis, multiple rounds of demyelination and associated inflammation may be required for axonal loss. Axonal loss in optic neuritis has been long appreciated clinically as optic atrophy and loss of the nerve fiber layer, and experimental models of optic nerve inflammation can be used to elucidate how this axonal damage occurs. It is likely that one major effect of inflammation is on the axonal cytoskeleton, as witnessed by changes in microtubule and neurofilament organization, and by changes in axonal transport.
In cases of acquired loss of myelin, for example, in idiopathic optic neuritis, there is abnormal conduction because of the changes in resistive and capacitive properties of the membrane brought about by demyelination. This can happen via loss of the compact morphology of the myelin alone, i.e., without frank demyelination. A low density of internodal sodium channels in adult optic nerve may allow some conduction after demyelination, as in optic neuritis. Axonal conduction becomes slowed, however, and in some cases may be blocked (“conduction block”), both resulting in decreased visual function. Even in a completely demyelinated axon, a low density of internodal sodium channels in demyelinated optic nerve may still allow conduction. Another phenomenon peculiar to demyelination is worsening of vision with heat or exercise, or Uhthoff’s phenomenon . Increased temperature and exercise are thought to decrease the sodium channel open-time during depolarization, resulting in less charge entering the axon, and a decreased likelihood that an adjacent section of demyelinated axon will be able to depolarize enough to cause opening of its own voltage-sensitive sodium channels. This leads to temperature-sensitive conduction block.
Optic neuritis has been modeled in rat and mouse models of experimental autoimmune encephalomyelitis, in which the immune systems of rodents are stimulated to react against myelin-associated proteins. Immunization against myelin-oligodendrocyte glycoprotein (MOG) induces multiple sclerosis–like demyelination throughout the brain including in the optic nerve. However, creating transgenic mice with T-cells directed against MOG or passively transferring anti-MOG T-cells in rats creates demyelinating disease largely confined to the optic nerve, mimicking optic neuritis. In such models, RGC axons are incidentally severed and fail to regenerate and, as after optic nerve trauma, RGCs die with a 1- to 2-week delay.
Compressive optic neuropathy
Compression of the optic nerve is a common clinical correlate of a large number of optic neuropathies. Besides the obvious causes such as neoplasms and aneurysms, the optic nerve can also be compressed by enlarged extraocular muscles (as in Grave’s ophthalmopathy), edema (as seen with indirect traumatic optic neuropathy, in which the nerve is contused within the optic canal), or optic disc drusen. Compression intrinsic to the nerve itself may occur in some forms of glaucomatous optic neuropathy in which increased IOP may cause bowing out and shifting of the lamina cribrosa, constricting the bundles of optic nerve axons within the lamina cribrosa pores. Although all three layers of meninges are present in the intraorbital and intracanalicular optic nerve, only the pia continues along the intracranial optic nerve. The clinical relevance of this is that a tumor arising from the optic nerve meninges themselves, (i.e., an optic nerve sheath meningioma), will normally continue through the optic canal but then extend along the sphenoid bone, and not along the course of the intracranial optic nerve or optic chiasm. This means that it is extremely rare for an optic nerve sheath tumor to extend toward the chiasm and thereby affect the other side. The more common way for optic nerve sheath meningiomas to become bilateral is by directly extending along the sphenoid bone meninges.
The effects of chronic experimental compression of the intraorbital optic nerve were delineated at the microscopic and ultrastructural level in a classic series of experiments by Clifford-Jones and colleagues. They found demyelination initially, followed by remyelination, even while the axons were still compressed. There were relatively minor findings of direct axonal loss. Demyelinated axons or the direct effects of pressure might be expected to lead to conduction block, which would be reversible. This could therefore explain the remarkable return of visual function after removal of tumors compressing the optic nerve.
Glaucoma
The most common optic neuropathy is glaucomatous optic neuropathy, distinguished by a distinct morphology of progressive excavation of the nerve head without significant pallor of the remaining neuroretinal rim. Within the retina, there are decreased numbers of RGC bodies in glaucoma, and this likely reflects death by apoptosis. The number of RGCs lost correlates with the visual field deficit. In addition to the RGC body loss, there is loss of the ganglion cell axons, manifested by segmental loss of the nerve fiber layer, increased cup-to-disc ratio, thinning of the optic nerve and chiasm, and changes in postsynaptic cell counts within the LGN (the main target of RGC axons in higher animals) and even the cerebral cortex.
A wide variety of pathologic evidence suggests that the optic disc is the primary site of injury. The focal areas of optic nerve axonal injury correlate with the focal areas of cell body loss in the retina and visual field defects. Thus the most typical glaucomatous visual field defects spread in an arcuate pattern but stop at the horizontal meridian in the nasal field. This site corresponds to the temporal raphe of the nerve fibers within the retina. The reason for this is that while there is a small distance between adjacent RGCs across the temporal raphe, the axons that correspond to these RGCs are separated by a large distance at the optic disc. In those cases when defects are seen on both sides of the horizontal meridian, it can be seen that spread has occurred in both the superior and inferior visual fields, but not directly across the meridian.
What mechanisms induce RGC death in glaucoma? Glaucoma has been extensively modeled in animals (reviewed in ). Studies of tissue from human patients with glaucoma and nonhuman primates and other mammals with experimental glaucoma confirm changes at the optic nerve head, such as with respect to bowing out of the lamina cribrosa, intra-axonal accumulation of organelles (consistent with blocked axonal transport; see Fig. 28.6 ), and Wallerian degeneration distal to the lamina cribrosa. Whether owing to mechanical trauma of axons, ischemia, generation of nitric oxide, or other causes, axonal injury causes changes in RGCs, eventually resulting in death. Increased IOP perturbs rapid anterograde and retrograde axonal transport at the lamina cribrosa. This may cause RGCs to be deprived of neurotrophic factors or other survival signals produced by brain targets.

Papilledema
Papilledema is defined as optic disc edema secondary to elevated intracranial pressure, and should not be used nonspecifically to denote disc edema. Pathologic examination of optic nerve heads with papilledema reveals intra-axonal edema, consistent with abnormalities of axonal transport. It is unclear whether the visual loss associated with chronic papilledema results from disturbances of axonal transport, or from ischemia owing to congestion of the optic nerve head, but the premise that an imbalance between the intracranial pressure and eye pressure contributes to glaucomatous injury has gained support from both animal and human data.
Retinal ganglion cell death after optic nerve injury
Death of RGCs is the final common pathway underlying virtually all diseases of the optic nerve, including glaucomatous optic neuropathy, anterior ischemic optic neuropathy, optic neuritis, and compressive optic neuropathy. In many diseases affecting the RGC axons (e.g., glaucoma or arteritic ischemia), the visual loss is permanent, reflecting the fact that RGC loss is irreversible. In some cases (e.g., chronic compressive optic neuropathy, acute optic neuritis, or papilledema) the visual loss can be reversed when the axonal damage is relieved, presumably because RGC death has not yet occurred. Even in glaucoma, there is now evidence that there is some period of RGC electrophysiological dysfunction and visual field defects that precedes frank cell death. However, if allowed to continue, these disorders can result eventually in permanent RGC death.
Apoptosis
A variety of techniques have been used to show that the death of RGCs after axotomy occurs by apoptosis. Pathologic studies show condensation of the nucleus and cytoplasm, budding of the cytoplasmic membrane, cleavage of DNA into 180 to 200 bp fragments, fragmentation of the cell into membrane-bound bodies, and heterophagy of these bodies. The apoptosis program is an intricate and sequential activation of multiple intracellular enzymes, including cysteine-dependent aspartate-directed proteases (caspases) and endonucleases regulated from the mitochondrial membrane by the Bcl-2 family of proteins. Proapoptotic family members such as Bax, Bak, and Bok are held in check by antiapoptotic members such as Bcl-2 and Bcl-xL. A third group in this family, including Bim and Bad, cannot activate apoptosis by themselves but can facilitate apoptosis by interfering with Bcl-2 or Bcl-xL’s ability to regulate Bax, for example. If antiapoptotic proteins are unable to balance the proapoptotic family members, either because they are sequestered by Bim-like proteins or are in short supply due to inadequate production, the anti-apoptotic proteins will increase mitochondrial membrane permeability and enhance release of cytochrome C into the cytoplasm. Cytochrome C binds to an adaptor protein Apaf-1, forming a complex that can initiate a cascade of enzymatic degradation of cellular components by a family of enzymes called caspases, beginning with the activation of procaspase-9. Also released from the mitochondria, a protein called Smac/Diablo binds to and inactivates an antiapoptotic protein called Inhibitor of Apoptosis (IAP), which normally represses activation of procaspases. Thus by at least two mechanisms, mitochondrial permeabilization by Bax inclines the balance toward apoptosis. Once the apoptosome is formed from the factors released from the mitochondria, the activation of caspases takes place. These in turn activate other caspases, and this cascade of events results in the activation of other enzymes within the cell that results in the breakdown of DNA and other macromolecules, membrane-bound compartmentalization of cell components, and then phagocytosis by adjacent cells.
Besides axonal injury, several other modes of cell death may induce apoptosis. For example, the binding of tumor necrosis factor to tumor necrosis factor receptors may induce apoptosis via death domain signaling and subsequent activation of caspase-8, initially bypassing the caspase-9 activation by the mitochondria. Glutamate excitoxicity may also induce apoptosis, and RGCs are particularly susceptible to high levels of glutamate in the extracellular space. It has been proposed that excitotoxic RGC death may explain pathologic conditions such as glaucoma. The evidence supporting this is that antagonists of glutamate excitotoxicity mediated by NMDA receptors generally decrease RGC death after optic nerve injury, and that there may be elevations of glutamate levels within the eye after optic nerve injury, or experimental or human glaucoma. Changes in NMDA receptor subunit expression follow axonal injury and may underlie changes in susceptibility to excitotoxicity. Variability in the sensitivity of RGCs to excitotoxicity in animal models, and the failure of clinical efficacy of NMDA receptor inhibition for prevention of progression in glaucoma, however, raises significant questions about this mechanism.
Time course
The rate and timing of RGC death after optic nerve injury depends on the species, age, RGC body size, and subtype of RGC, and the distance from the site of injury to the RGC. For example, after optic nerve injury in goldfish and frogs, ganglion cells do not die, but hypertrophy and regenerate axons within 1 to 2 months. Conversely, in adult rodents, optic nerve injury leads to initiation of cell death within 5 to 7 days, and 50% to 90% cell death occurring within weeks to months. Similarly, ganglion cells from squirrel and owl monkeys die approximately 4 to 6 weeks after axotomy. In humans with chiasmal compression for at least 6 months, there is extensive loss of ganglion cells from the nasal hemiretinas; yet even 35 days after transection of the ipsilateral optic tract there is sparing of some temporal ganglion cells. In contrast, neonatal RGCs are far more sensitive to axotomy than adults, perhaps because naturally occurring developmental cell death is taking place at the same time. As an example, in postnatal rodents, 90% of RGCs die within 48 hours of optic nerve crush.
There are many subtypes of RGCs, which can be defined by differences in dendrite morphology, target field innervation, electrophysiology, visual stimulus response properties, and gene expression. Different subtypes of RGCs show varying susceptibility to death after optic nerve injury in animal models of trauma or glaucoma. Originally this was discovered in animal models using measures of cell size, with data suggesting that large RGCs with alpha-type physiology die sooner after optic nerve insult. These data were called into question as it was not technologically easy at the time to assess whether cells changed morphology or physiology after injury, for example, to distinguish whether large RGCs died or atrophied to appear smaller, or similarly whether RGCs changed their physiology or response patterns after axon injury. More recent use of in vivo imaging and tracking of individual RGCs, as well as single cell RNA sequencing of RGCs over a time course after injury, have improved on these formative observations. Together these data suggest that RGCs do indeed change in size, response physiology, and gene expression after injury, confirming some of confounding results of the older data, but also suggesting that different subtypes of RGCs are indeed differentially susceptible to axon injury and death. Furthermore, limited data from human electrophysiology measurements in patients with glaucoma, for example, confirm that some of the same observations from animal models are seen similarly in human disease, such as preferential loss of OFF-pathway compared with ON-pathway RGCs.
It is controversial whether the response of ganglion cells to axotomy depends on the distance between the lesion and the cell body. Some studies have shown that the shorter that distance, the more rapid the degeneration and the more severe the effect. This could be due to lack of a trophic signaling along a length of residual axon or more rapid transmission of a signal mediating cell death, with either mechanism possibly mediated through retrograde axonal transport. Others have suggested that the timing of ganglion cell degeneration is not correlated with the distance to the lesion. Axotomized fibers from the macula degenerate later than those more peripheral, suggesting that the distance from the site of axotomy to the retina does not affect timing of RGC death. Differences in ganglion cell survival also depend on the site of optic nerve transection in relation to the vascular supply of the retina. If the ophthalmic or central retinal arteries are transected, then the inner retina, including the ganglion cell layer, will be infarcted, confusing interpretation of RGC survival.
Signaling of axonal injury
How does axonal injury result in induction of apoptosis? RGC death after optic nerve injury or target removal appears to take place through several mechanisms, including lack of neurotrophic factors from the target tissue, excitotoxicity from physiologic or pathologic levels of glutamate, free radical formation, leakage of cellular constituents from the end of the axon, proliferation of macroglia, activation of microglia, buildup of excess retrogradely transported macromolecules, and breakdown of the blood-brain barrier. Even the contralateral retina may be affected by a unilateral optic nerve injury, suggesting the involvement of intrachiasmal signaling or retinopetal fibers.
RGCs are highly dependent on neurotrophic factors during development and in the adult for their survival. Responsiveness to neurotrophic factors also appears to require physiologic levels of electrical activity. If the optic nerve is injured and CNTF or other neurotrophic factors are introduced into the vitreous or retina, there is increased survival of RGCs compared with control eyes. It is possible that the increased survival seen after neurotrophin administration is not a specific rescue effect from neurotrophin deprivation, but rather a generalized prosurvival effect. Neurotrophic factor restoration and electrical activation have moved into clinical trials in recent years with some suggestion of positive effect, but much work remains to be done to identify dosing and delivery regimens with consistent and meaningful effects.
Phagocytosis and immune activation
Optic nerve injury also leads to activation of local glial cells and recruitment of immune cells. For example, during Wallerian degeneration, resident microglia, as well as circulating monocytes, phagocytose myelin debris, although there may be a contribution from oligodendrocytes and astrocytes. Probably most phagocytes in the degenerating optic nerve are derived from the circulation, although not all studies are in agreement. Deficiencies in the activation of microglia may lead to decreased clearance of myelin debris, and subsequent inhibition of axonal regeneration ; conversely, increasing macrophage-mediated clearance of debris can increase regeneration. Broadly speaking, interfering with microglial and macrophage recruitment and activation is very effective in preventing RGC death in animal models of optic neuropathies.
More recent data has strongly implicated innate immune regulation of RGC death in glaucoma models. Innate immunity is driven by many components of genetics and environment, including exposure to pathogens and formation of the gut commensal microflora (called the microbiome). Microbiome effect on neurodegeneration by way of regulation of innate immunity is now gathering support from both human research subjects and animal models. For example, in mice raised in clean environments where the relative lack of diversity in the microbiome does not stimulate innate immune recognition of certain epitopes, elevating IOP does not lead to ganglion cell death, whereas elevating IOP in mice raised in normal environments does. The link to innate immunity is demonstrated by the observation that transfer of CD4+ T-cells from the latter to the former mice leads to IOP-induced ganglion cell degeneration.
Astrogliosis
The role of optic nerve astrocytes in the pathologic processes of gliosis is complex. As a reaction either to local injury or to remote death of adjacent axons, astrocytes are observed to hypertrophy and extend processes, partly as a type of scar formation, but likely also as part of the process of recruiting or modulating other cell types. Recent data suggest that some astrocytic responses lead to neuronal death whereas other astrocytic responses lead to adaptive or even neuroprotective effects. Such so-called neurotoxic and neuroprotective astrocytes, broadly referred to as A1 and A2 astrocytes, likely represent a useful if oversimplified construct. Astrocyte activation after injury may also explain in part the failure of ganglion cell axons to regenerate after injury (see further).
Failure of axon regeneration
In adult mammals, there is essentially no axon regenerative response after optic nerve injury. This contrasts with lower vertebrates—for example, not only do goldfish RGCs survive after their axons are transected, but they are also able to extend neurites and ultimately establish correct connections with their targets. Research in the early 20th century showed that mammalian RGCs, like other centrally projecting neurons, make only abortive attempts to regenerate their axons. Even when conditions permit axonal extension, only a small minority of RGCs eventually extend their axons. For example, severed rodent RGC axons approach the optic nerve head but then reverse and meander in another direction. Analysis of the proximal stump of the transected optic nerve just posterior to the optic nerve head shows early regenerating axons, which then die off.
Thus in most cases, optic nerve injury and axon loss leads to permanent vision loss. Our understanding of the mechanisms preventing axon regeneration in adult mammals continues to advance, particularly with respect to the role of inflammation, discussed previously, and of glial-associated inhibitory signals, and neuron-intrinsic limitations to axon repair, discussed further.
Glial inhibition of neurite extension
Since the early experiments of Aguayo and colleagues, it has long been known that RGCs and other CNS neurons regenerate axons into peripheral nervous system (PNS) grafts (e.g., sciatic nerve), but not into CNS tissue. Because RGCs do not extend axons in the absence of specific extracellular signals (discussed previously), regenerative failure might be explained in part by a relative inability of mature CNS astrocytes and oligodendrocytes to secrete trophic signals after injury. Evidence has supported this hypothesis, but it turns out that astrocytes and oligodendrocytes also actively inhibit axons from regenerating. The nonpermissive nature of the CNS (but not PNS) substrate for axonal elongation is therefore of great interest and has resulted in a large number of candidate molecules. The most studied inhibitory molecules are components of oligodendrocyte myelin and proteoglycans expressed by astrocytes, and these molecules and their signaling mechanisms have been reviewed recently in detail.
Resident microglia and/or macrophages recruited to the site of injury may also inhibit or fail to support axon growth. Although macrophages/microglia do increase in number at the site of injury, they may not be appropriately activated for support of axonal extension, and may differ from other macrophages in fundamental ways. If activated macrophages/microglia are poorly able to phagocytose degraded myelin, the inhibitory signals found in myelin may prevent axonal regeneration.
Neuron-intrinsic limitations to axon regeneration
Interest has also focused on the possibility that failure of regeneration reflects a specific inability of an adult RGC to extend its axon because embryonic axons are able to do so when transplanted into adult tissue. Recent research has focused on determining whether the rate and extent of axon growth depends purely on extracellular signals and substrates, as discussed previously in regard to optic nerve development, or also on the intrinsic state of the neuron. Elsewhere in the CNS, embryonic neurons can regenerate their axons quite readily, but they lose their capacity to regenerate with age. This developmental loss of regenerative ability has generally been attributed to the maturation of astrocytes and oligodendrocytes, and to the production of myelin, all of which strongly inhibit axon growth after injury. In experiments in which the inhibitory environment is removed or molecularly blocked, however, only a few percent of axons regenerate, and functional recovery typically proceeds remarkably slowly (discussed further). For example, RGCs take 2 months to regenerate through a peripheral nerve graft.
These experiments indicate that the neurons themselves are partly responsible. For example, axons from postnatal day 2 or older hamster retinas lose the ability to reinnervate even embryonic tectal explants. When cultured in the complete absence of CNS glia, embryonic RGCs extend their axons 10 times faster than postnatal or adult RGCs. Interestingly, the decrease in the axon growth ability of RGCs occurs sharply at birth during the period of target innervation, but target contact may not be responsible for this change. Rather, retinal maturation, and possibly a membrane-associated signal from retinal amacrine cells, may be sufficient to induce the developmental decrease in RGC axon growth ability. Once this signal is sent, the loss is permanent—removal of the amacrine cells does not allow the RGCs to speed up again. This may explain the failure of RGC regeneration, at least in part—even in a perfect environment, RGCs may lack the intrinsic capacity to rapidly grow their axons again, after they have done it once during development.
What molecular changes underlie the developmental loss in rapid axon growth ability? RGCs could gradually increase expression of genes that limit axon growth or decrease expression of genes necessary for faster axonal elongation, or both. For example, recent data has pointed to a family of transcription factors that may regulate axon regenerative ability in RGCs. Cytoplasmic kinases or phosphatases such as Ca 2+ -dependent signaling kinases and calmodulin kinases (CaMKs) may also provide a downstream mechanism for axon growth control. Second messengers such as cAMP and cGMP have also been implicated in the intrinsic axon regenerative control of the RGC, perhaps by modulating responsiveness to positive neurotrophic factors or to myelin-associated axon growth inhibitors. Finding the molecules that, when modified, truly enhance functional regenerative repair remains a major ongoing goal in optic nerve research today.
Optic nerve repair
The major goal of any optic nerve repair strategy must be to protect or regenerate the connections of RGC axons to their targets in the brain, as these connections are ultimately what serve vision.
Neuroprotection and retinal ganglion cell survival
A first step to protecting optic nerve axons from a degenerative process is preventing RGC death. Neuroprotection, first proposed for other CNS diseases, has been considered in recent years as a possible treatment for optic neuropathies. There is a variety of laboratory evidence using cell culture or animal models of optic nerve disease to suggest that one or more neuroprotective methodologies eventually may be successful in clinical disease, but thus far no strategy has been successfully proven in large, randomized controlled clinical trials in human patients with glaucoma. A number of clinical trials are now in progress and it is likely that small molecule or gene therapy approaches will prove neuroprotective in the coming years.
Regeneration of retinal ganglion cell axons
The same factors that strongly promote RGC survival also tend to strongly promote RGC axon regeneration, with few exceptions. Furthermore, our understanding of the mechanisms of axon growth has generated much progress in understanding regenerative failure. As mentioned previously, reversing the developmental decrease in intrinsic axon growth ability could also be leveraged to promote axon regeneration. Targeting the immune system, retinal or optic nerve cell metabolism, or astrogliosis could also prove effective strategies. A combinatorial approach may be required—both the intrinsic neuronal growth state and the extrinsic environment may have to be optimized for successful regeneration after injury. A major challenge to translating axon regenerative therapies to the clinic, however, will be the length of time it takes for axons to grow down the lengthy optic pathways to their targets in the brain and create and refine new connections to their postsynaptic partners. This longer period will imply that long clinical trials may be required—a barrier to translation in chronic disease.
Optic nerve remyelination
In the case of optic neuritis, where the primary defect in RGC function is attributable to oligodendrocyte demyelination, strategies to enhance remyelination are paramount. Currently, the major approach involves treating patients with steroids, which presumably interferes with the ongoing inflammatory insult and allows a faster rewrapping of optic nerve axons and return to baseline vision. In the presence of demyelination, oligodendrocytes may reconstitute themselves and remyelinate axons, although the nature of the inducing chemical signals has not been well characterized. Similarly, chronic treatment with immune modulators decreases the risk of subsequent demyelinating events. Cell transplant therapy with oligodendrocytes or progenitor cells may prove fruitful in such disease, as has been explored for multiple sclerosis.
“Neuroenhancement” of retinal ganglion cell function
Before RGCs have died, and even before their axons are fully damaged in the optic nerve and would require a proregenerative therapy, RGCs may be partially inhibited in the optic nerve from their full functional capacity. For example, glaucoma causes dysfunction and then death of RGCs, but the window between dysfunction and death in humans is not currently known. Acute studies of IOP raising and lowering in humans and chronic studies of IOP raising and lowering in animal models both suggest that a window between dysfunction and death exist in this degenerative disease. Thus, factors known to enhance RGC health and, ultimately, survival could be applied to intervene before death and potentially reverse dysfunction, here termed “neuroenhancement.” These remain major goals for the biomedical community for patients with glaucoma.
Neuroenhancement has been used in the literature either generically to refer to improvement of axon regeneration, or more specifically to improvement of cognitive function in degenerative brain disease. Compared to the many years it would take a glaucoma trial to demonstrate neuroprotection (e.g., one would ideally demonstrate that progression of visual field losses is slowed or halted), it may be possible to measure an enhancement of RGC structure or function acutely. A potential “neuroenhancement” therapy would target RGCs that are dysfunctional and/or atrophic. With the same neurotrophic factors that stimulate neuroprotection in the long term, such dysfunctional RGCs could hypertrophy to their normal size, or resume normal electrical responsiveness or optic nerve transmission of visual information, or both. Novel approaches to measuring RGC structure and function, referred to as optic neuropathy biomarkers , will likely prove critical to advancing such therapeutic candidates through early phase clinical trials.
Conclusions
The optic nerve is the critical conduit for visual information to pass from the eye to the brain. The past decade has seen dramatic increases in our fundamental understanding of optic nerve physiology and pathophysiology, particularly at the cellular and molecular levels, but much remains to address the many optic neuropathies that lead to vision loss and blindness. The next decade will likely prove even more exciting, as advances in regenerative medicine begin to transition from the bench to the clinic.
Acknowledgements
Supported by the National Eye Institute (P30-EY026877) and an unrestricted grant from Research to Prevent Blindness, Inc. The author is indebted to Leonard Levin for the foundations of this chapter carried from prior editions.
References
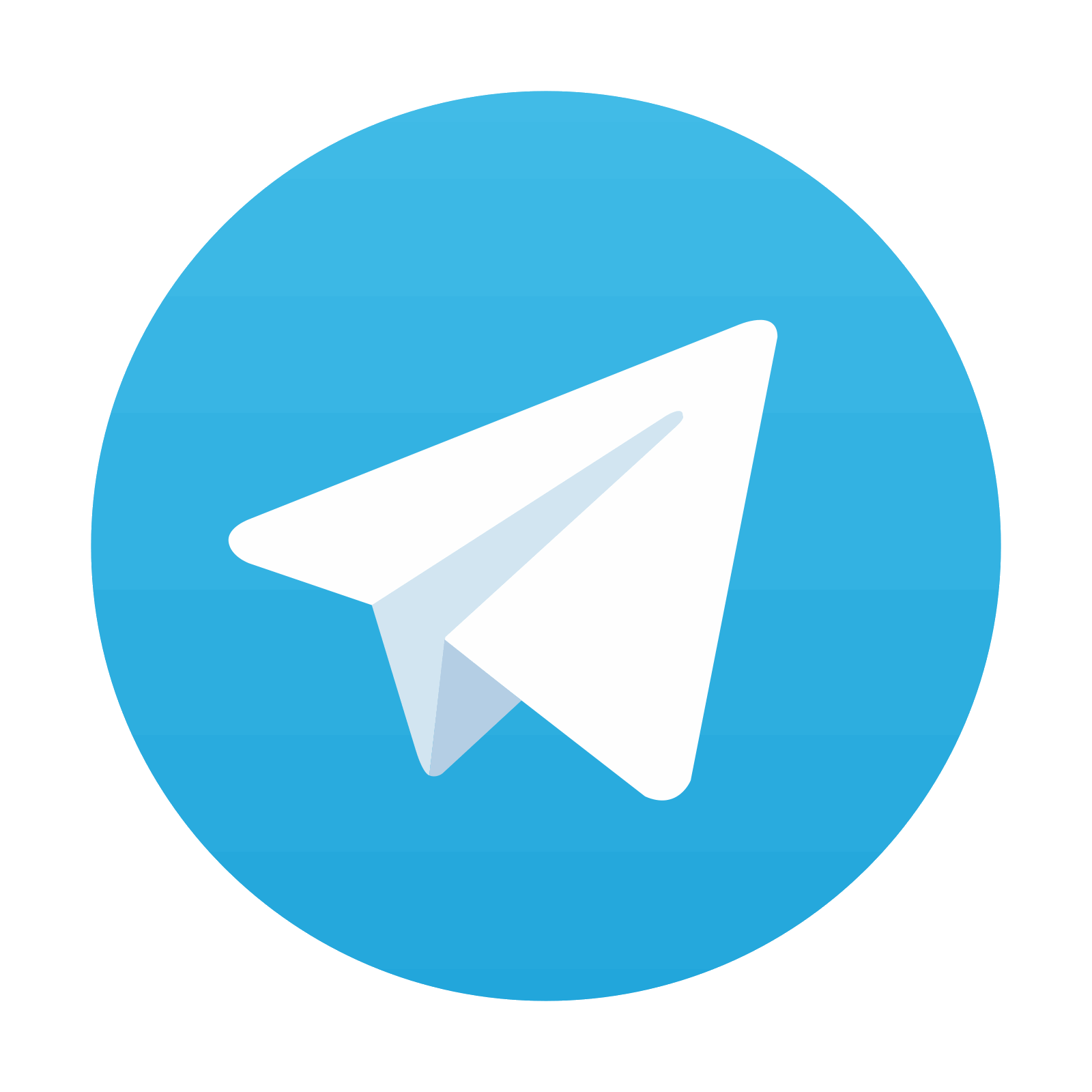
Stay updated, free articles. Join our Telegram channel
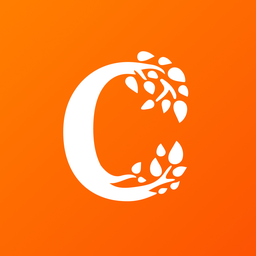
Full access? Get Clinical Tree
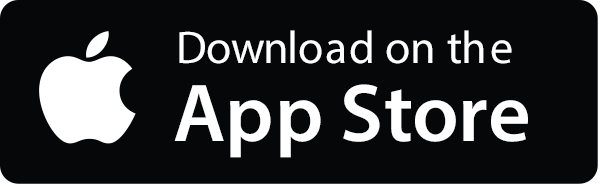
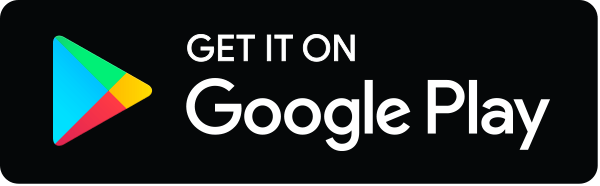
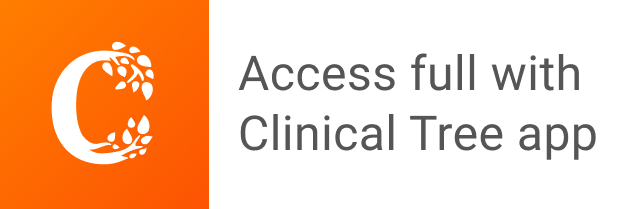