The vascular system of the eye
The vascular system transports oxygen and metabolites in the human body, ensures thermoregulation of the body and its organs, and is responsible for waste material removal. The driving force of blood is the heart, which pumps the blood through the body and ensures a pressure gradient from the arterial to the venous system. Blood vessels include three fundamental components: arteries, veins, and the microvasculature, including arterioles, venules, and capillaries. Within the arterioles, pulsatile flow is converted into steady flow. This comes with a pronounced drop in pressure, and as such the arterioles are the main site of vascular resistance.
The human posterior pole of the eye is nourished by two independent vascular beds that receive their inputs from branches of the ophthalmic artery (OA). The inner retina, including the retinal ganglion cells and the retinal nerve fiber layer, is supplied by the retinal circulation. The outer retina does not contain any blood vessels and is supplied by the choroid via diffusion of oxygen through the retinal pigment epithelium (RPE).
The retinal vessels get their vascular supply from the central retinal artery (CRA), which is a branch of the OA. It is located within the dural sheath of the optic nerve and enters the optic disc through the lamina cribrosa. The diameter of the CRA is in the order of 150 to 500 μm. Hence, the CRA and its branches are functionally arterioles, constituting the primary site of vascular resistance for the retinal circulation. In the literature on ocular vasculature and blood flow, these vessels are, however, usually referred to as arteries, and the current chapter follows this terminology. The CRA branches into smaller vessels over the internal surface of the retina, which can be seen ophthalmoscopically, and finally into terminal arterioles and capillaries, which can be visualized with technologies such as optical coherence tomography angiography (OCTA) ( Fig. 11.1 ). In 25% of humans, a cilioretinal artery can be found and mostly supports the macular region.

Retinal arteries are surrounded by a capillary-free zone that can be visualized by OCTA and adaptive optics scanning laser ophthalmoscopy (AO-SLO). In nonhuman primates this zone is between 30 and 70 mm, depending on the eccentricity ( Fig. 11.2 ). The tissue surrounding the larger retinal branch arteries is oxygenated directly from these vessels. The system of the retinal veins shows similarities with the arterial angioarchitecture, and the central retinal vein (CRV) runs in parallel to the CRA, draining blood into the cavernous sinus. In contrast to other vascular beds, the retina does not contain precapillary sphincters. Hence, the retinal capillaries show continuous perfusion. The retinal capillary network is arranged in four layers ( Fig. 11.3 ). The superficial plexus is located in the nerve fiber and ganglion cell layer and contains the larger retinal branch arteries and veins, as well as microvessels, including a dense capillary network. The intermediate plexus contains capillaries in a lobular arrangement that shows considerable tortuosity. They are located in the inner nuclear layer (INL) among the amacrine cells. The deep vascular bed consists mainly of capillary vessels and is located at the outermost side of the INL. The radial peripapillary capillaries are located in the nerve fiber layer close to the optic nerve head (ONH), where the nerve fiber bundles are thick and have high metabolic demand to maintain ion pumping.


Depending on the eccentricity, retinal vessels have one to seven layers of smooth muscle cells. The retinal veins have a basement membrane, and the walls contain some pericytes. The capillaries of the retina show a high density of pericytes. Traditionally a ratio of 1:1 between pericytes and endothelial cells has been reported. A recent study of the human retina, utilizing ultrastructural criteria, showed a 94.5% frequency of pericyte coverage on human retinal capillaries. Endothelial cells are lined in parallel to the blood vessels. The blood vessels share their basement membrane with pericytes and endothelial cells ( Fig. 11.4 ).

The choroidal circulation gets its main vascular supply from the long and short ciliary arteries and is part of the uveal system. The choroid consists of five layers, including Bruch’s membrane and three vascular layers, as well as the suprachoroidea. The outermost vascular layer is Haller’s layer, which includes the largest choroidal arteries and veins. Sattler’s layer contains smaller arteries and veins. Anatomically there is no clear border between Haller’s layer and Sattler’s layer. The choriocapillaris consists of highly anastomosed capillaries and is between 7 and 10 μm thick. These capillaries are fenestrated and show a diameter that is larger than the diameter of the retinal capillaries.
The ONH has its own vascular supply that shows significant interindividual variability in humans. This surface layer of the ONH is, in most humans, supplied by the CRA and part of the retinal vascular system. In cases with a cilioretinal artery, the corresponding sector of the surface layer usually gets its blood supply from this vessel. Below the surface nerve fiber layer is the prelaminar region, which spans toward the lamina cribrosa. The deeper layers are the vascular supply of the lamina cribrosa and the retrolaminar regions. All of these vascular beds receive their blood supply via the posterior ciliary arteries (PCAs) and the peripapillary choroid. In many cases the medial and lateral short PCAs form the circle of Zinn and Haller around the optic nerve, which can be seen ophthalmoscopically in some subjects ( Fig. 11.5 ).

The vasculature of the anterior segment gets it main supply from the anterior ciliary arteries. The cornea is not vascularized in healthy humans. The limbus, however, contains a ring of vasculature that contributes to corneal oxygenation. Whereas the conjunctiva is richly vascularized, the sclera contains only a few blood vessels because of its low metabolic demand. The anterior uveal system includes the iris and the ciliary body. The principal blood supply of the iris is the major arterial circle, which enters the iris through its root. The iris blood vessels are radially oriented. The ciliary body is also supplied by the major arterial circle, which branches into the anterior parts of the ciliary processes and forms a capillary bed.
Oxygenation and blood flow of the retina
There are fundamental differences in the oxygenation of the inner and outer retina. The outer retina is avascular, and the photoreceptors depend on oxygen supply from the choroid. The inner (including the two nuclear layers, the two plexiform layers, and the nerve fiber layer) retina receive oxygen mainly from the retinal vessels. Oxygen profiles in the retina differ between light- and dark-adapted conditions. In the dark-adapted retina, a trough in oxygen tension (PO 2 ) is seen at approximately 80% retinal depth in a variety of species ( Fig. 11.6 ). This is related to the high oxygen consumption of photoreceptor inner segments. As gradients in the PO 2 curve from both sides can be observed, both the choroidal and retinal circulation contribute to photoreceptor oxygenation, with the majority of oxygen diffusing from the choroid. In the light-adapted retina, the retinal oxygen profile looks different ( Fig. 11.6 ). Whereas there is little change in choroidal PO 2 , the minimum PO 2 in the outer retina is higher than in the dark-adapted retina. The contribution of the retinal circulation to photoreceptor oxygenation is minimal or completely absent in the light-adapted retina.

In the inner retina the average PO 2 in the deeper region is slightly lower than in the very superficial region in dark-adapted animals. This difference between the deeper region and superficial region disappears when the retina is light adapted, most likely because some choroidal O 2 diffuses from the choroid toward the inner retina during this condition. In contrast to the outer retina, inner retinal PO 2 is almost independent of the illumination level of the retina. Because the oxygen consumption of the vitreous is low, vitreal PO 2 close to the retina is a good indicator of inner retinal PO 2 , although this may not be true in pathologic conditions such as diabetic retinopathy (DR).
The photoreceptors also consume glucose via anaerobic glycolysis, leading to a considerable accumulation of lactate. In contrast, anaerobic glycolysis is absent in the inner retina. Under light-adapted conditions, the photoreceptors are solely oxygenated by the choroid. In darkness, however, the PO 2 reaches 0 mmHg at the proximal side of the inner segments, which means a change in the gradient of PO 2 toward the inner segment. This implies that in darkness, some of the oxygen to the photoreceptors is delivered from the retinal circulation. Photoreceptors consume a significant amount of oxygen that is mainly used for maintenance of the dark current and the generation of guanosine triphosphate.
Akin to any other vascular bed, blood flow in the eye is determined by ocular perfusion pressure (OPP) and vascular resistance (R). As such, volumetric blood flow can be written according to the Hagen-Poiseuille equation as Q = OPP/R. R increases linearly with length of the vessel segment and the viscosity of blood and is inversely related to the fourth power of the radius of the vessel.
Retinal blood flow is characterized by a high vascular resistance and accordingly blood flow rate is low. Using noninvasive technology, the retinal blood flow rate has been measured to be approximately 40 μL/min in healthy subjects. The diameter of larger retinal branch veins and arteries can be up to 200 μm, which makes the vessels, functionally, venules and arterioles. There is an almost linear relationship between retinal vessel diameter and retinal blood velocities in both arteries and veins, as expected from Murray’s law ( Fig. 11.7 ). Blood velocities in retinal arteries are, however, higher than in veins, because of the equation of continuity. Blood velocities in larger retinal vessels are in the order of 6 to 25 mm/s. In the capillaries, velocities are in the range between 1 and 4 mm/s and exhibit quite significant spatial and temporal variations. Recently, resistance and blood flow in the retina have been modeled using retinal vessel calibers, fractal dimension, perfusion pressure, and population-based hematocrit values. Retinal blood flow decreases with age, most likely owing to an age-related loss of retinal ganglion cells. Oxygen extraction in retinal vessels is accordingly low. The arteriovenous oxygen difference is high, in the order of 35% to 45%. Total retinal oxygen extraction is accordingly in the range of 2.0 to 2.5 μL (O 2 )/min. Choroidal blood flow is much higher than retinal blood flow because the vascular resistance is low. In humans, there is no technique to measure absolute choroidal blood flow. Data in nonhuman primates indicate that the absolute flow rate is approximately 600 μL /min. In humans, pulsatile ocular blood flow has been estimated as 600 to 1000 μL/min, but the ratio between pulsatile and nonpulsatile flow is unknown. The arteriovenous O 2 difference cannot be measured in humans, but it is considered to be very small, with a value of approximately 1%, as measured in cats. There is some evidence for gender differences in ocular blood flow, but the evidence for this is inconclusive.

Techniques for measuring blood flow
Measurement of blood flow in the human eye is not an easy task. Multiple techniques have been developed, but none has gained widespread clinical application for measuring blood flow. In recent years, OCTA and laser speckle flowgraphy have gained some popularity. Some of the older historical methods will only be mentioned briefly.
Invasive technology in experimental animals
Microsphere technology
The microsphere method for measuring blood flow can be used in multiple organs, and absolute values of regional blood flow can be obtained. When microspheres are injected into the systemic circulation the number of entrapped microspheres is proportional to the blood flow through the tissues. In the eye, plastic radioactive microspheres and fluorescent microspheres, as well as colored microspheres, have been used. Whereas in principle many organs can be studies simultaneously, reliable measurements of local blood flow require optimization of the size and number of microspheres. This also makes it difficult to study retina and choroid in the same experiment, because of the differences in capillary lumen. As with all other methods in experimental animal studies, care has to be taken such that the anesthesia and experimental procedures do not influence the results. This can either be directly because of the effects of the anesthetic drugs on vascular tone and/or vascular regulation, or via the fluctuations of arterial blood pressure during the course of the experiments. An obvious advantage of the microsphere method is the lack of surgical intervention. However, the animals cannot be followed longitudinally because the technique is terminal.
Hydrogen clearance
Hydrogen clearance is based on the detection of the rate of clearance of an inert gas from the tissue of interest. The technique has been used in the eye by several investigators to study perfusion in different tissues. The technique is, however, not easily applied to the eye because the condition that the tissue is perfused uniformly in a three-dimensional (3D) volume is neither fulfilled for the choroid nor the retina. Hydrogen can either be administered via lung inhalation, intra-arterial injection or local routes. The hydrogen is detected via an intraocularly placed microelectrode that allows for the measurement of arrival and clearance of the gas. Placement of the microelectrodes can be done in proximity to either larger retinal vessels or vascularized tissue, resulting in different clearance curves. The blood flow values are usually reported in values of mL/min per 100 g tissue.
Oxygen-sensitive electrodes
Techniques that quantify oxygen can be divided into those that quantify the partial pressure of free oxygen in tissue (PO 2 ) and those that measure the saturation of oxygen bound to hemoglobin (SaO 2 ). SaO 2 and PO 2 are interrelated in a nonlinear way and can be interconverted except at conditions of very high oxygenation, when SaO 2 is almost 100%. Oxygen-sensitive electrodes work based on polarography. When a noble metal is polarized at about −0.7 V relative to a reference electrode, it becomes a cathode, where oxygen is chemically reduced. This is associated with a current that is proportional to the PO 2 in the tissue. Different oxygen electrodes have been used in the vitreous humor, the retina, and the ONH. This technique provides high spatial resolution for measuring tissue PO 2 .
Noninvasive technology in humans
Dye-based angiography
Fluorescein and indocyanine green angiography are important tools for visualizing blood flow in clinics. There have been multiple approaches to quantifying retinal and choroidal blood flow based on this technique. The most advanced technique is to combine video fluorescein angiography based on scanning laser ophthalmoscopy with digital image processing. Early studies quantified retinal perfusion by dye dilution technique, but time resolution was low, recording images at a frame rate of 1 to 2 Hz. Parameters obtained were arm-retina time and the arteriovenous passage time, but only semiquantitative measurements of perfusion were obtained. More advanced approaches use arteriovenous passage time and mean dye velocity using the early phase of the angiograms. Another approach is to directly visualize retinal capillary microcirculation in perifoveal capillaries, providing information on the macular microcirculation. Whereas dye-based angiography still has an important role in clinical decision-making, quantification of blood flow based on the technology has lost importance. The invasive nature of dye administration and the potential risks associated with it make it difficult to apply the technology for research purposes.
Measurement of retinal vessel diameters
Measurement of retinal vessel diameter or retinal vessel caliber has been widely used as a biomarker for both ocular and systemic disease. The technique has the advantage that it can be extracted from any high-quality fundus images. Parameters that are reported include central retinal arterial equivalent (CRAE), central retinal venular equivalent (CRVE), and arteriovenous ratio ( Fig. 11.8 ). Several formulas for summarizing retinal vessel diameters have been proposed. A commercial fundus camera–based system is available, specifically targeting retinal vessel caliber analysis, as well as the response of retinal vessels to flicker light stimulation. In terms of blood flow, the information is limited, as no information on retinal blood velocity is available. In patients with systemic hypertension, for instance, CRAE is reduced, indicating arteriolar vasoconstriction, but CRVE is increased, most likely due to inflammation. Whether hypertension is associated with altered volumetric blood flow based on these measurements is, however, unclear. Owing to the equation of continuity, it is, however, obvious that the ratio of retinal blood velocity between arteries and veins is shifted toward higher values, because the retina is an end organ and total arterial blood flow needs to be equal to total retinal blood flow.

Laser Doppler velocimetry
The technique is based on the optical Doppler effect. Laser light that hits moving erythrocytes is shifted in frequency, and this frequency shift is dependent on the velocity of the scatterers. The first measurements in retinal vessels were performed in the 1970s to measure the blood velocity. As the technique does not provide any depth resolution, a continuous spectrum of Doppler shifts is detected because of the velocity profile within the vessel. The maximum Doppler shift corresponds to the maximum flow velocity in the center of the vessel. Absolute measurement of retinal blood velocity is, however, difficult because the angle between the laser beam and the retinal vessel needs to be known. One way to overcome this problem is to detect the scattered laser light in two distinct directions, a technique called bidirectional laser Doppler velocimetry. Combining this technology with measurement of vessel diameter provided the first results for total retinal blood flow in humans.
Laser Doppler flowmetry
The technique is related to laser Doppler velocimetry, but in contrast to single blood vessel, vascularized tissue is illuminated. Based on a scattering theory of light in tissue, average blood velocity, blood volume, and blood flow can be derived. The technology has been used to study ONH and subfoveal choroidal blood flow in humans. No absolute values of blood flow can be obtained and, as such, the method is mainly suitable for measuring blood flow changes in response to stimuli. The sampling depth of laser Doppler flowmetry is difficult to determine in vivo. Studies in nonhuman primates indicate that laser Doppler flowmetry technique samples blood flow changes mainly in the superficial layers of the ONH and is less influenced by perfusion in deeper regions. When the laser beam is directed toward the fovea, the signal is evidently coming from the choroidal microvasculature, because the retina lacks vessels in this region. When the laser beam is directed toward other retinal locations, the signal is also influenced largely by the choroidal perfusion. Mapping of the microcirculation of the human retina can be achieved by scanning laser Doppler flowmetry based on a scanning laser ophthalmoscope. Laser Doppler flowmetry has also been used by various groups in experimental animals and much of our knowledge on blood flow regulation in the eye stems from these experiments.
Color Doppler imaging
This technique is based on the acoustical Doppler effect. It combines B-scan ultrasonography for structural imaging with velocity extraction based on the Doppler shift of the wave. In the eye this technique is mainly used for the visualization of blood velocities in the following retrobulbar vessels: OA, PCAs, and the CRA, as well as other vessels, including retrobulbar veins ( Fig. 11.9 ). The typical frequencies of the ultrasound probes are in the order of 5 to 10 MHz to achieve sufficient penetration. Doppler shift of the ultrasound wave does not only depend on the blood velocity but also on the Doppler angle. The direction of the sampling gate therefore needs to be adjusted based on the anatomy of the artery. Whereas this is usually achieved without problems in the CRA and the OA, it is more difficult in the PCAs. Peak systolic and end diastolic velocities (PSVs, EDVs) are extracted from the time slope of the Doppler shift. Mean flow velocity (MFV) can be calculated as the time mean of the spectral outline over a heart cycle. In addition, a resistance index (RI = [PSV – EDV]/PSV) can be calculated as a measure of vascular resistance distal to these vascular beds. A study in healthy subjects indicates, however, that this is a poor measure of vascular resistance in the retina. The technique is not capable of measuring volumetric blood flow because the resolution is not sufficient to resolve the vessel diameter with the required precision. As the ultrasound probe is put on the closed eye lid, care has to be taken such that the intraocular pressure is not increased due to the force induced on the eye.

Laser speckle flowgraphy
Laser speckle flowgraphy measures blood flow using laser speckle statistics. Laser speckles arise from reflection of coherent light at rough surfaces. The speckle pattern that appears under the illumination of laser irradiation can only be described statistically. When objects are moving, for instance, red blood cells in the blood stream, the structure of the pattern varies. The higher the variation, the higher the velocity of the scatterers. Early approaches using laser speckle flowgraphy only allowed for semiquantitative analysis of perfusion. Later, instruments were developed that allow for quantification of blood flow at the posterior pole of the eye. Nowadays several commercial systems are available.
In most systems a near infrared laser is used as a light source, coupled to a fundus camera and a digital charge–coupled device camera. The most widely used output parameter of laser speckle technology is mean blur rate (MBR), which is a measure of relative blood flow velocity and is expressed in arbitrary units (au). When a scan is started, 118 images are captured at a rate of 30 frames per second, resulting in a measurement time of 4 seconds. From the 118 acquired images, a color-coded map is calculated, which depicts the distribution of perfusion.
The system can either be used to study perfusion in vascularized tissue or to study blood flow in retinal vessels. For perfusion measurements, the ONH area is selected by positioning an ellipsoid region of interest at the ONH margin. Thereafter, larger retinal vessels are automatically detected by thresholding according to their higher blood velocities. Thus, MBR can be determined for the total ONH area, for vessel area, or for tissue area ( Fig. 11.10 ).

There is a longstanding discussion whether the technique measures flow, velocity, or some quantity related to flow. It is obvious that the speckle contrast in the tissue is not only affected by the velocity of the red blood cells but also by the number of moving scatterers. The technique has been validated in a variety of animal studies and it has been shown that measures of laser speckle flowgraphy correlate well with values obtained using either the microsphere method or the hydrogen gas clearance method. As with laser Doppler flowmetry, the penetration depth of the technique is not completely understood. When the laser is directed toward vascularized tissue of the retina, a sum signal between retinal and choroidal blood flow is measured. Animal data indicate that the retinal contribution is in the order of 10%, which is in agreement with data from laser Doppler flowmetry studies. The results may be influenced by pigmentation and, as such, results between different ethnicities may not be comparable. The majority of data with this technology have been obtained in Japan, and recently the technique was also successfully applied in Caucasians. When measurements were done in retinal vessels, the correlation with data as obtained with Doppler OCT was not good, particularly at higher arterial flow rates because of saturation effects.
Doppler OCT
Doppler OCT is a functional extension of OCT. The first description of this technique was realized based on a time domain–OCT system. In Fourier domain–OCT, the Doppler shift of light can be quantified by analyzing the phase of the complex OCT signal. Similarly, laser Doppler velocimetry measurement of blood velocity requires knowledge of the incident angle of the laser beam. Multibeam systems for the illumination of the retina were realized to overcome this angle ambiguity. Combining these measurements with diameter measurements of retinal vessels by either using fundus photography or phase tomograms of OCT allows for measurement of total retinal blood flow. Other approaches to overcome the angle ambiguity were also realized. The use of bidirectional Doppler cross sections perpendicular to the illumination plane allows for calculation of retinal blood flow independent of the Doppler angle. Dual-plane scanning patterns have also been used to determine the angle between blood flow and the scanning beam. When using 3D Doppler data sets, en-face images can be used to extract absolute blood velocity, as well as total retinal blood flow, without knowledge of the Doppler angles ( Fig. 11.11 ). Another approach is to study retinal vessels at relatively high Doppler angle, which is automatically detected by autoalignment. Recently it has been proposed to use the forward-scattering signal instead of the backward-scattering signal, because it is insensitive to vessel orientation.

Doppler OCT has been also used to reconstruct the velocity vector field from measured phase data, allowing for the visualization of velocity profiles at retinal bifurcations ( Fig. 11.12 ). Line scanning protocols of multichannel OCT were employed to investigate venous pulsatile caliber oscillations and flow pulsatility.

OCT angiography
OCTA is a technique that is closely related to Doppler OCT. The first approach to noninvasively visualizing the vasculature in the human eye based on OCT was based on Doppler phase, as well as variance or power of the phase analysis. Later, multiple algorithms and scan protocols were proposed based on logarithmic intensity and speckle contrast imaging. Split spectrum amplitude decorrelation (SSADA) improves signal to noise and bulk eye motion artifacts. Optical microangiography (OMAG) is another approach that includes both the amplitude and the phase of the OCT signal.
Nowadays, repeated B-scans are used to detect motion contrast, thereby visualizing the ocular vasculature ( Fig. 11.13 ). Nonmoving tissues will not produce an autocorrelation signal in repeated B-scans but moving objects produce a signal that is dependent on the blood speed. Based on this mode of function it is obvious that the technique is sensitive to eye motion and motion artifacts are common. Segmentation of OCT angiograms is usually done to extract either two or three retinal vascular plexuses, as well as the choriocapillaris ( Fig. 11.14 ).


OCTA has also been applied to the anterior segment of the eye. The technique has several advantages over other vascular anterior segment imaging modalities including rapid image acquisition, noninvasiveness, absence of leakage, 3D visualization, and imaging in cases of corneal opacification. The technology has been used to study the vasculature of the cornea in pathologic conditions, the iris, sclera, episclera, and conjunctiva.
OCTA has recently renewed the interest of many clinicians in ocular blood flow because it is readily available as a functional extension of OCT platforms. The technology allows for the noninvasive visualization of the vasculature with unprecedented 3D resolution. This has led to the discovery of novel insights into the pathophysiology of retinal and choroidal vascular disease, and also to the standard use of the technology in clinical care. A variety of quantitative metrics have been proposed for the analysis of OCT angiograms. The foveal avascular zone (FAZ) can be separately analyzed for each capillary plexus. The vessel density is based on the skeletonized vessel map and is calculated as the ratio of vessel area to image area. A one-pixel line represents the vessel, thereby excluding the effect of vessel diameter. Perfusion density is calculated as the ratio of the binarized perfusion area to the entire imaged area. Alternative metrics such as fractal dimension or complexity index were also calculated from OCT angiograms.
Since the choriocapillaris is highly vascularized, the nonperfused area is usually used as an outcome measure after binarization of the image. These nonperfused regions are called flow voids, flow deficits, or signal voids. The size and number of such flow voids can provide information on choroidal integrity. The B-scan rate, the A-scan rate, the scan direction, and the oversampling ratio, as well as the lateral and axial resolution, need to be considered when calculating vascular metrics based on OCTA. Magnification is another important factor to consider. The field of view is a function of axial eye length, and several approaches were published on how to correct for this. This obviously has a major effect on quantification of FAZ size, which in turn affects measures such as perfusion density and vessel density.
Whenever quantitative metrics are calculated it is important to understand the area that is being analyzed. Generally, data for vessel density or perfusion density are not comparable between different OCT systems, because each vendor uses its own algorithm. There is currently no consensus on an optimal approach for this problem.
Spectroscopic oximetry
Noninvasive retinal oxygen saturation measurements were first done in 1965 based on absorption profiles of hemoglobin that depend on SO 2 . This technique has been improved by using digital imaging and automated software solutions. Currently, there are two commercial systems for noninvasive spectroscopic oximetry on the market, both using a two-wavelength approach. These two images are taken concomitantly so that the influence of eye movements is eliminated ( Fig. 11.15 ). Validation of this technique is not easy, because of the lack of a gold standard method. One approach to study the accuracy is to compare SO 2 values obtained using oximetry in retinal arteries with systemic SO 2 values in patients with systemic hypoxemia. The two-wavelength approach may, however, be influenced by retinal pigmentation and vessel diameter and therefore requires careful calibration. Application of such calibration values to eyes from different ethnicities is, however, not possible because of differences in pigmentation levels. Another factor influencing the results is blood velocity. To overcome these limitations, several authors have used multiwavelength or hyperspectral approaches for retinal oximetry. Alternatively, white light OCT can be used to study retinal SO 2 , a technique that has also been extended toward the retinal microcirculation. Monte Carlo models have recently been developed that dealt with the impact of scattering, blood volume fraction, and lens yellowing and will hopefully improve the reliability of this technique in the future.

Regulation of ocular blood flow
Local metabolic control
Metabolic control of blood flow is based on the interaction between parenchymal cells and the smooth muscle cells. In the retina and the ONH, the key regulator is oxygen. During conditions of hypoxia, vasodilator signals are activated to increase retinal perfusion. In both animals and humans, this increase in perfusion is, however, not associated with an increase in inner retinal oxygen consumption as long as the systemic PO 2 is above 35 mmHg. Little is known about the mechanisms of oxygen-induced vasodilation in the retina, but nitric oxide (NO) and prostaglandins appear to be involved in this process. During hyperoxia, strong retinal vasoconstriction is observed, which is associated with a pronounced reduction in blood flow ( Fig. 11.16 ). OCTA metrics also decrease during hyperoxia, but it needs to be kept in mind that the technique does not measure blood flow, but parameters such as vessel or perfusion density. The vasoconstrictor response to hyperoxia involves endothelin-1, as well as the arachidonic acid metabolites thromboxane and 20-HETE. In contrast to blood flow in the retina, perfusion in the choroid is almost independent on systemic PO 2 . This is associated with large amounts of oxygen diffusing from the choroid into the retina, increasing inner retinal PO 2 .

Perfusion in both the retina and the choroid is strongly dependent on partial pressure of carbon dioxide ( Fig. 11.16 ) (PCO 2 ). In the brain it has been demonstrated that reduced extracellular pH is mainly responsible for vasodilation in response to local changes in PCO 2 . In the retina this has not been confirmed, but the most likely effect is the diffusion of CO 2 across the blood-retinal barrier, which results in reduced pH in the extracellular space. Activation of acid-sensing ion channel-1A by extracellular acidosis seems to be a key mechanism in CO 2 -induced vasodilation in the brain. The cerebral blood flow response in the brain is dependent on nitric oxide (NO), a mechanism that has also been confirmed for the ocular vasculature.
The metabolic theory of blood flow regulation assumes that blood flow is adapted via a feedback loop in response to changes in PO 2 , PCO 2 , pH, and other vasoactive mediators. This may, for instance, play a role in hypoxic states, such as early diabetes when retinal blood flow increases. It is, however, unlikely that such metabolic mechanisms play an important role in blood flow regulation during changes in arterial blood pressure or neuronal stimulation, because of their relatively slow mechanisms of action.
Autoregulation in response to changes in perfusion pressure
Autoregulation refers to the ability of a vascular bed to maintain its blood flow during changes in perfusion pressure. In its strict sense, it refers to isolated vascular beds. Changes in vascular tone during in vivo experiments are not only due to the change in perfusion pressure but also due to potential changes in the neural or humoral input. This is unlikely to occur in the retina as the retinal vasculature lacks autonomic innervation. In the choroid, however, the vessels are richly innervated and in vivo responses to pressure stimuli are at least partially medicated via neural mechanisms.
Another issue relates to the calculation of perfusion pressure. This is defined as the difference in arterial minus venous pressure. In the retina neither arterial nor venous pressure can be calculated noninvasively in vivo. Noninvasive plethysmographic measurements of retinal venous pressure have been performed, but the results are dependent on cerebrospinal fluid pressure. Hence, the vast majority of autoregulation studies have estimated OPP as mean arterial pressure (MAP) – intraocular pressure (IOP) in the supine position and as two-thirds MAP – IOP in the sitting position to account for the drop in pressure between the level of the eye and the level of the heart. Although this may be a good approximation, there are several limitations to this approach. On the one hand the arterial tree from the heart to the eye represents a vascular resistance, which is associated with a drop in pressure. Hence, the arterial pressure at the level of the CRA will be lower than at the level of the heart. On the other hand, retinal venous pressure is slightly higher than IOP, to allow blood to exit the eyeball toward the venous circulation. The same also holds true for the choroidal veins. As such, the calculated OPP will always overestimate the actual OPP by a couple of mmHg. There are two principal methods to change OPP, either via modification of the arterial side or the venous side, or a combination of both. Multiple techniques have been used to study this behavior. The majority of studies investigated autoregulation either during an increase in blood pressure or an increase in IOP.
Myogenic mechanisms play a key role in autoregulation. Myogenic tone is defined as vasoconstriction that occurs in an isolated blood vessel at a constant pressure. Similar to the brain, retinal arteries respond to changes in transmural pressure, which is defined as the pressure within the vessel minus outside the vessel, with development of myogenic tone. When arterial blood pressure is decreased, myogenic tone is reduced and vessel diameter increases. An increase in blood pressure induces and increases myogenic tone, thereby reducing vascular diameter. These changes in vessel diameter are associated with changes in vascular resistance that keep blood flow constant within certain limits of OPP.
With increases in blood pressure, a reduction in vessel diameter helps to normalize wall tension. This concept is supported by the fact that similar phenotypic changes are seen in isolated vessels studied under pressurized conditions in vitro, but where blood flow is absent. The vascular myogenic response is intrinsic to vascular muscle cells, and experiments in cerebral vessels have shown that the mechanism is still functioning when endothelial cells are removed. The mechanisms regulating myogenic response in brain and retina seem to be closely related. When transmural pressure increases, cation channels on the surface of the vascular smooth muscle cells are activated in response to the mechanical stress. This leads to cell membrane potential depolarization, which induces vasoconstriction owing to the increase in voltage-dependent Ca influx. Transient receptor potential channels contribute to myogenic signaling in the retinal vasculature, with TRPV2 being the most important regulator. While myogenic mechanisms in vascular smooth muscle cells are intrinsic to vascular muscle, additional factors related to endothelial-, metabolic-, neural-, and immune-related signaling, have also been identified.
A schematic drawing of autoregulation in response to changes in OPP is shown in Fig. 11.17 . Two examples are shown, one in which autoregulation is present over an OPP range of 40 mmHg and another in which the autoregulatory range is lower, only spanning over an OPP range of 20 mmHg. The autoregulatory range is also termed autoregulatory plateau. The lower limit of autoregulation is defined at the OPP value at which blood flow starts to decline. The OPP value at which blood flow starts to increase when OPP is increased is called the upper limit of autoregulation. This autoregulatory capacity is achieved by the adaptation of vascular tone during changes in transmural pressure, as discussed previously in this section. It is often stated that the upper and lower limit of autoregulation represent maximum vasoconstriction and maximum vasodilation, respectively. Experimental evidence, however, has shown that even below the limit of lower autoregulation, retinal vessels still exhibit flicker-induced vasodilation.

Failure of autoregulatory capacity may have severe clinical consequences. When the OPP falls below the lower level of autoregulation, tissue will be at risk for ischemia and hypoxia. When the OPP exceeds the upper level of autoregulation, there is a risk of bleeding. Several common eye diseases are associated with abnormal autoregulatory ranges, which are considered to play a role in their pathophysiology.
In the retina, autoregulation has been proven in a wide variety of studies and most likely reflects myogenic blood flow regulation. During an isometric exercise–induced increase in OPP, retinal blood flow in humans stays constant until an increase in the order of 30% to 40%. Retinal blood flow autoregulation has also been shown to occur when OPP is decreased, for instance, during an experimental increase in IOP ( Fig. 11.18 ).

In older textbooks it is frequently mentioned that the choroid is a strictly passive vascular bed with a linear pressure-flow relationship. This is to large degree based on animal experiments using the microsphere technology. Newer studies have, however, proven that the pressure-flow relationship is nonlinear in vivo ( Fig. 11.19 ). In humans, the choroid has been shown to react to both a decrease and an increase in OPP. Animal studies support the concept that myogenic mechanisms play a role in this response, but there is currently no study available investigating myogenic tone in choroidal arterioles. A strong modulating function of neural components to this response has been proven, which may be responsible for most of the vascular tone changes during perfusion pressure challenges.

As mentioned previously, the vascular anatomy of the ONH is complex. Little is known about potential differences between the superficial and deep vascular components of the ONH in term of pressure autoregulation. Most of the data on ONH autoregulation used either laser Doppler flowmetry or laser speckle flowgraphy, and therefore data are limited to the superficial vascular bed. With this technology, autoregulatory behavior was seen during an increase, as well as a decrease, in OPP. Two studies have compared autoregulatory changes in the ONH and choroid during standardized stimuli by either using laser speckle flowgraphy or laser Doppler flowmetry and found distinct differences between the two vascular beds ( Fig. 11.20 ).

An important difference to autoregulation in the brain is that in the eye, venous pressure can become very high when IOP is high. There is now evidence from a wide array of animal and human studies that blood flow reacts differently if either the arterial or the venous pressure is modulated ( Fig. 11.21 ). In humans, the regulation of choroidal and ONH blood flow during combined changes in systemic blood pressure and IOP is dependent on the absolute values of blood pressure (BP) and IOP. Blood flow in both vascular beds is less sensitive to changes in arterial blood pressure than in IOP. Interestingly, this finding is compatible with a myogenic mechanism being involved. During an artificial increase in IOP, venous pressure will increase with an associated increase in the transmural pressure gradient. As mentioned earlier, the myogenic theory is based on the concept that changes in transmural pressure cause smooth muscle relaxation when OPP is lowered. Hence, ChBF may exhibit much lesser autoregulatory response during an IOP-induced drop in OPP, because it is paralleled by a decrease in perfusion pressure gradient. When OPP is changed via the arterial side, however, the full myogenic response is initiated due to the increase in transmural pressure. This concept is also supported by a study in healthy subjects in which the IOP was lowered pharmacologically with an associated increase in the autoregulatory plateau.

Several studies have found that autoregulation of ocular vascular beds is altered by pharmacologic intervention. A role of NO in autoregulation of all ocular vascular beds has been shown in a wide variety of animal and human studies ( Fig. 11.22 ). Interestingly, NO synthase inhibition alters the choroidal but not the ONH pressure-flow relationship when IOP is increased, again highlighting the differences in the mechanisms between choroid and ONH. Modulating the endothelin system has also been proven to change the ocular pressure/flow relationship, mediated via the endothelin A receptor ( Fig. 11.23 ). Other factors that have been shown to modulate the autoregulatory pressure response in the eye include adenosine, NMDA receptors, α- and β-adrenergic blockade, and calcium channel blockade.


In a study of perfused and pressurized brain slices, it was postulated that astrocytes are involved in modulating myogenic tone during changes in pressure. This concept is supported by rabbit experiments in which animals treated with the gliotoxic L-2-aminoadipic acid (LAA) showed reduced autoregulatory reserve in the ONH.
Neurovascular coupling
When neurons are stimulated with light the retinal vessels vasodilate. This response is due to a fundamental mechanism, termed functional hyperemia, that describes the increase in blood flow when neurons are stimulated. This phenomenon has been described in detail in the brain but also occurs in the retina. The increase in blood flow and vessel diameter during flicker stimulation has been described by many authors in both animals and humans ( Fig. 11.24 ). This is paralleled by an increase in retinal oxygen extraction. This relaxation can either be mediated via smooth muscle cells or via the retinal pericytes that also show contractile tone in the retina.

It has been shown that NO and prostaglandins are involved in the process of neurovascular coupling in the brain. Results for NO have also been confirmed in the retina. Whereas these substances are produced in neurons and directly diffuse to the vessels, another mechanism plays a key role in brain neurovascular coupling. Astroglial cells act as intermediaries in neurovascular signaling. According to this model, astrocytes stimulated via neurotransmitters such as glutamate promote the production of ATP in neurons ( Fig. 11.25 ). This induces an increase in intracellular Ca 2+ , which activates phospholipase A2 and other enzymes to produce vasodilators that mediate the increase in blood flow. In addition, endothelium-derived factors appear to contribute to the signaling that is responsible for functional hyperemia.

In the retina, the principal glial cell is the Müller cell, which also has an important role in mediating neurovascular coupling. Blockade of the neuron to glia signaling causes a pronounce reduction of the response to light stimulation, thereby highlighting the importance of this mechanism. The increase in intracellular Ca 2+ leads to a release of vasoactive substances from Müller cells that include the vasodilators prostaglandin E2 and epoxyeicosatrienoic acids, as well as the vasoconstrictor 20-hydroxy-eicosatetraenoic acid, resulting in net vasodilation under physiologic conditions. The main role of NO seems to be the regulation of production of these vasodilators in glial cells.
During systemic hyperoxia, the hyperemic response in the retina is preserved in the rat, but increased in humans. Another potential mechanism involved in neurovascular coupling is the release of K + from Müller cells. Nonetheless, potassium ion concentration increased in the vitreous humor close to the ONH as measured with microelectrodes during flicker stimulation in cats. However, K + siphoning does not play a role in functional hyperemic response in the retina.
Recently, nanotube-like processes that connect two pericytes with retinal capillaries systems were found to play a role in neurovascular coupling in the retina ( Fig. 11.26 ). These processes have an open-ended proximal side and a closed-ended terminal that connects the pericyte processes via gap junctions. This allows intracellular Ca 2+ signaling to mediate communication between adjacent pericytes. Pericytes rely on the processes to control neurovascular coupling. A role for caveolin-1 in flicker-induced retinal vasodilation was also established. Caveolin-1 knockout mice show reduced response of ONH blood flow to flicker stimulation ( Fig. 11.27 ).


Endothelial factors
The endothelium plays a key role in regulating vascular tone. Arteries, arterioles, capillaries, venules, and veins are lined with endothelial cells. Endothelial cells produce both vasodilators and vasoconstrictors that regulate blood flow. Endothelial dysfunction as observed in diseases such as diabetes alters retinal blood flow and contributes to macro- and microvascular damage. Many mechanisms contribute to regulation of vascular tone by endothelial cells. These can be roughly divided into two main components: release of signaling molecules that modify vascular tone and spread of electrical signals from endothelial cells to smooth muscles.
The most important endothelium-derived vasodilator is NO. NO is produced from the amino acid L-arginine via three isoforms of NO synthase (NOS), NOS1, NOS2, and NOS3. NOS1 and NOS3 are constitutive, and NOS2 is inducible, playing an important role in pathologic states that are associated with alteration in the NO system. NO is a small gaseous mediator that can easily diffuse through tissue similar to oxygen. As NO cannot be stored in vivo, it only affects the cells that are in close proximity to the production site. A wide variety of studies have proven that inhibition of NO synthase reduces basal vascular tone in the ocular vasculature ( Fig. 11.28 ). NO has a wide array of functions in the vasculature of choroid and retina, as well as ONH, and appears to be involved in vascular dysregulation as observed in some diseases. Other endothelium-derived vasodilators that affect vascular tone include potassium ion and endothelium-derived hyperpolarizing factors that have not been identified so far.

Three structurally different endothelin isoforms, endothelin-1, endothelin-2, and endothelin-3 have been identified, with endothelin-1 being one of the most potent vasoconstrictors known. Circulating levels of endothelin-1 are low, and its main role is to act as an autocrine/paracrine mediator. Endothelin-1 is a potent endothelium-derived vasoconstrictor that controls vascular tone in many organs, including the eye. The vascular effects of endothelin-1 are mediated by tow receptor subtypes called endothelin A and endothelin B receptors. In the eye, endothelin A receptors are localized at blood vessels, whereas endothelin B receptors are mainly found on neural and glial tissues. The endothelin A mediates vasoconstriction, and stimulation of the endothelin B receptor induces vasodilation ( Fig. 11.29 ).

Arachidonic acid metabolites control vascular tone via multiple metabolites including prostaglandins, thromboxane A2, and prostacyclin. The majority of receptors that mediate the vasoactive actions of the arachidonic acid metabolites are present at the posterior pole of the eye. Ocular levels of prostanoids are higher in the perinatal than in the adult eye indicating an important role of prostaglandins in the regulation of blood flow in newborn animals. As such, prostanoids appear to be involved in the hypoxic processes that lead to retinopathy of prematurity. In the newborn animal, prostanoids play a role in autoregulatory processes. In adults, this effect may be less pronounced, but prostaglandin E2 is produced during an increase in perfusion pressure, partially mediating autoregulatory vasoconstriction. This is, however, controversial because some studies have reported that prostaglandin E2 acts as a potent vasodilator in both the retina and the choroid. Controversial data have also been reported for prostaglandin E1 and prostaglandin F2α. Similar to other vascular beds, thromboxane is a potent vasoconstrictor.
Shear stress regulates endothelial function, thereby influencing vascular tone. In large systemic arteries, flow-mediated vasodilation is well established. In smaller vessels, this phenomenon is more difficult to measure because of the lower shear stress levels owing to lower blood velocities and smaller vessel diameters. Techniques to measure flow velocity such as laser Doppler velocimetry allow for calculation of shear stress in retinal arteries based on theoretical velocity profiles. Although Doppler OCT technologies allow for measurement of velocity profiles, this technology has not yet been used to study velocity profiles. High shear stress, as caused by increased retinal blood velocities, compromise the inner blood-retinal barrier function, thereby establishing a link between perfusion abnormalities and leakage as seen in diabetes.
Neural control of blood flow
Owing to the rich innervation of the choroid by parasympathetic, sympathetic, and trigeminal sensory nerves, choroidal blood flow is under neural control. Facial parasympathetic nerves regulate choroidal blood flow, as shown by several animal studies. Mediators such as NO, acetylcholine, and vasoactive intestinal polypeptide (VIP) all cause vasodilation in the ocular vasculature. It has, for instance, been shown that administration of VIP causes an increase in choroidal blood flow. Activation of preganglionic input to the pterygopalatine ganglion by facial nerve stimulation increases choroidal blood flow. Direct activation of the superior salivatory nucleus also induces choroidal vasodilation and an increase in blood flow. Centrally mediated autonomic reflexes also play a role in the change of choroidal vascular resistance during changes in blood pressure Fig. 11.30 .

The choroid is also innervated by sympathetic noradrenergic nerve fibers that originate from the superior cervical ganglion. The sympathetic neurotransmitter noradrenaline induces pronounced vasoconstriction in the choroid. Cervical sympathetic stimulation causes an increase in choroidal vascular resistance. The sympathetic input appears to exert a small vasoconstrictor tone in the choroid under basal conditions. The sympathetic input into the choroid may also regulate choroidal vascular tone during elevated blood pressure, to keep blood flow constant. This is supported by experiments showing that superior cervical ganglion removal impairs choroidal autoregulation during changes in perfusion pressure. Sensory nerve fibers from the trigeminal ganglion also innervate the choroid, and have been proposed to be involved in the thermoregulation of choroidal blood flow.
Humoral control of blood flow
Adenosine is a nucleoside which is present in the entire body and exerts strong vasodilator effects. Animal studies have proven that adenosine increases blood flow in newborn piglets, as well as in adult cats. In humans, adenosine increases retinal and choroidal blood flow. The nucleoside is involved in hypoxia-induced vasodilation and retinal autoregulation in newborn animals, but does not modulate the choroidal pressure/flow curve in the human choroid. Recent evidence suggests that the vasoactive effect of adenosine triphosphate differs in vessels at different branching levels.
Glucose has important vascular effects impairing endothelium-dependent relaxation. In the eye, hyperglycemia induces vasodilator effects in the retina and the choroid. In other vascular beds, this vasodilator effect has been attributed to changes in osmotic load. Alternatively, the vasodilator responses to hyperglycemia may be linked to an increased ratio of NADH/NAD+, because of an increased rate of reduction of NAD+ to NADH. In the eye, insulin also causes vasodilation which is additive to glucose vasodilator action. The increase in choroidal blood flow is reduced after NOS inhibition, and is enhanced by coadministration of L-arginine.
Altered blood flow in ocular disease
Glaucoma
Multiple cross-sectional studies have shown that glaucoma is associated with reduced retinal and ONH blood flow. There is a longstanding discussion whether this is cause or consequence of the disease. On one hand it has been argued that a loss of retinal ganglion cells and their axons will result in decreased oxygen demand and capillaries may die as a result of this reduced need. On the other hand, theories have been formulated suggesting that ischemia may promote apoptosis of retinal ganglion cells, thereby increasing the risk of visual field loss. Most likely both phenomena are true, because evidence has been accumulated for both mechanisms.
The first hypothesis has been supported by several recent studies indicating a strong spatial association between retinal perfusion and visual field defects ( Figs. 11.31 and 11.32 ). The second hypothesis is, however, nowadays supported by a wide variety of studies. Color Doppler imaging studies have shown that low blood velocities in the arteries supplying the ocular vasculature are associated with future Visual field loss does progress. These results were confirmed by other studies using either laser Doppler flowmetry, OCT angiography or laser speckle flowgraphy ( Fig. 11.33 ). Moreover, there is evidence that both high and low blood pressure are risk factors for glaucoma incidence and progression. Nocturnal hypotension is also a risk factor for visual field deterioration. Additionally, cardiovascular disease has been identified an important risk factor for glaucoma progression.



Evidence for altered autoregulation in glaucoma has been obtained from multiple studies. Group correlation between blood pressure or OPP and ocular hemodynamic parameters have been reported in multiple studies. This correlation is reduced after pharmacologic reduction of IOP, in agreement with the idea that IOP has a main influence on the pressure/flow relationship in the eye.
Multiple studies have reported abnormal blood flow responses to changes in OPP in glaucoma. These studies can be grouped into investigations in which OPP was reduced and those in which IOP was increased. Evidence has also accumulated that the response to changes in OPP shows a wide variability among glaucoma patients. A few studies did not find any difference in the autoregulatory behavior between glaucoma patients and healthy controls, but the change in OPP induced in these studies was most likely too small to reach the lower or upper limit of autoregulation. Abnormal autoregulation was also shown by a study investigating the variations of ONH blood flow during diurnal variations in OPP.
Altered autoregulation in glaucoma is linked to pericyte dysfunction and loss of interpericyte tunneling nanotubes. In addition, it has been shown that reduced ganglion cell thickness in healthy subjects is associated with both high blood pressure and abnormal blood flow autoregulation, which may make this group of subjects more susceptible to glaucomatous damage. This is also compatible with findings in a nonhuman primate model indicating that abnormal autoregulation is a consequence of ganglion cell loss.
A reduced hyperemic response to stimulation with diffuse luminance flicker has been consistently reported using several techniques to assess blood flow. This is compatible with results showing an abnormal oxygenation response to flicker stimulation in glaucoma patients. One study did not report a difference between flicker-evoked blood flow changes, as measured with laser speckle flowgraphy, in glaucoma patients compared with healthy controls, but the study was most likely underpowered. A longitudinal study did not find an association between reduced functional hyperemia and structural progression in glaucoma patients, but again the number of included patients and the duration of follow up was too low to draw any meaningful conclusions. Glaucoma has also been associated with endothelial dysfunction and changes in the endothelin system. Evidence for endothelial dysfunction has been obtained from systemic, as well as ocular, vascular stimulation tests. Dual inhibition of endothelin receptors increases ocular blood flow in patients with glaucoma. In patients with pseudoexfoliation glaucoma, an accumulation of pseudoexfoliative microfibrillar material leads to vascular endothelial dysfunction.
Diabetes and diabetic retinopathy
Retinal vascular changes are well documented in diabetes and DR, including dysfunction of the blood-retinal barrier associated with the development of diabetic macular edema. Other key features include vascular basement membrane thickening and a progressive loss of pericytes and vascular endothelial cells. During the course of this disease, vasodegeneration occurs, which is clinically seen as vascular dropout. This capillary dropout and the retinal ischemia triggers the release of growth factors including vascular endothelial growth factors (VEGF), which then leads to proliferative DR.
There are conflicting results of whether retinal blood flow is increased or decreased in early stages of DR or animal models of DR. This may be related to differences in the technology used to assess blood flow, but also due to different phenotypes. In this respect, it is important to mention that both insulin and glucose may influence the results, owing to their vasodilator actions.
OCTA has enabled the study of important aspects of vascular alterations in diabetes and DR. Features that can be studied with OCTA include microaneurysms, neovascularization, peripheral retinal nonperfusion, and FAZ alterations, as well as quantitative changes in perfusion and vessel density. Wide-field OCTA is nowadays the preferred method to identify peripheral capillary nonperfusion ( Fig. 11.34 ). Interestingly, wide-field OCTA has also been shown to provide improved detection of retinal neovascularization that is not detected clinically. Quantitative OCTA metrics predict the risk of DR progression and may therefore add to traditional risk factors in the management of DR.

OCTA can also be used to study choroidal changes in diabetes. Significant loss of choriocapillaris has been described histologically, but in vivo studies on choroidal blood flow in diabetes have been sparse. Analysis of OCTA slabs has again proven that pronounced vascular changes are present in the diabetic choroid. It has been shown that retina inflammatory processes appear to trigger endothelial cell damage in the choroid. Nevertheless, the role of the choroid and its blood flow in the development and progression of DR remains poorly understood.
Diabetes is characterized by abnormal retinal and ONH vascular autoregulation. The mechanism underlying this effect is not well characterized. Obviously, loss of perictyes, as well as endothelial dysfunction, are candidates for being involved in the loss of autoregulation. Uncoupling of gap junctions is also a candidate mechanism for disrupting the autoregulation.
Functional hyperemia is reduced in the diabetic retina in a stage-dependent manner ( Fig. 11.35 ). Correlation between reduced blood flow responses to flicker stimulation and endothelial dysfunction has been observed in diabetes. Interestingly, experimentally induced hyperglycemia also impacts neurovascular coupling in the retina, which is compatible with results showing reduced flicker-induced retinal vascular reactivity in subjects with impaired glucose tolerance. Abnormal retinal neurovascular coupling has also been observed in animal models of DR elucidating some of the mechanisms underlying this effect. Upregulation of NOS2 in retinal neurons and glial cells appears to play an important role in changing neurovascular coupling in diabetes. The mechanism for the NO-induced reduction in neurovascular coupling is believed to include the blockade of production of vasoactive factors released from Müller cells. This mechanism is supported by results showing that the NOS2 inhibitor aminoguanidine restores neurovascular coupling in the diabetic rat retina.
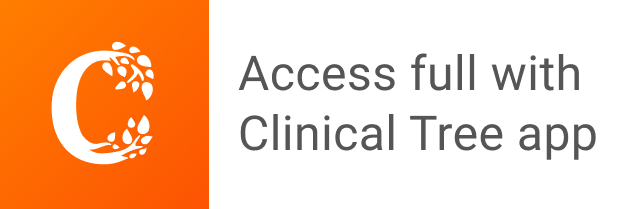