Introduction
The ocular circulation is unique and complex due to the presence of two distinct vascular systems, namely the retinal and uveal systems. The part of this circulation, the one that supplies the fundus of the eye, has the useful property that it can be observed using the ophthalmoscope, an optical instrument introduced by Helmholz in the middle of the 19th century. In the early 1960s, the fundus camera, a device based on the principle of indirect ophthalmoscopy, allowed the recording of the passage of fluorescein through the human retinal vascular system, from which Hickam and Frayser derived the first quantitative measurements of retinal hemodynamics. Technological developments in the field of optics and lasers have since led to a variety of non-invasive techniques, which have permitted the investigation of various parameters pertaining to human ocular hemodynamics and the response of these parameters to a number of physiologic and pharmacologic stimuli. These techniques have provided information on human ocular circulatory physiology and may help in understanding the role of ocular blood flow in the pathogenesis of eye diseases of vascular origin. Yet much of our basic knowledge of ocular blood flow regulation is based on data from animal experiments; thus, in the following, basic principles from animal experiments will be presented together with the human data available.
Anatomy of the ocular circulation
Knowledge of the anatomy and the regulatory mechanisms of the various ocular vascular beds is crucial for understanding the pathophysiologic changes occurring during the evolution of several systemic and local diseases threatening vision. The delivery of metabolic substrates and oxygen to the retina in higher mammals, including humans and other primates, is accomplished by two separate vascular systems, the retinal and the choroidal systems. In lower mammals, such as rabbit and guinea pig, the retinal vessels are present in only a small area of the retina or are lacking. In this case, the retinal metabolism depends almost completely upon the choroidal circulation.
The ocular vessels are all derived from the ophthalmic artery (OA), a branch of the internal carotid artery. The retinal and choroidal vessels differ morphologically and functionally from each other. The OA gives off two to three main ciliary arteries (CAs), i.e. the nasal and the temporal, which supply the corresponding hemispheres of the choroid via branches of the posterior CAs (PCAs) , and recurrent branches of the anterior ciliary arteries (ACAs). The ACAs arise from the extraocular muscular arterial branching from the OA. The central retinal artery (CRA) may branch from the same segment of the OA as the PCAs or from one of the main PCAs. , It enters the optic nerve from below, approximately 10–15 mm behind the globe, from where it assumes its central position in the optic nerve up to the optic disk ( Fig. 10.1 ).

Vascular supply of the retina
The retinal circulation is an end-arterial system without anastomoses. The CRA emerges at the optic disk where it divides into two major branches. These in turn divide into arterioles extending outward from the optic disk, each supplying one quadrant of the retina, although multiple branchings of the retinal arterioles towards the peripheral retina may occur. Retinal arteries and veins divide by dichotomous and side-arm branchings. The terminal arterioles branch off at almost right angles from the main vessel. In approximately 25 percent of human eyes, a cilioretinal artery emerging from the temporal margin of the optic disk supplies the macular region, exceptionally feeding the foveal region ( Fig. 10.2 ).

The larger vessels lay in the innermost portion of the retina, close to the inner limiting membrane. Their walls are in close spatial relationship with glial cells, mainly astrocytes ( Fig. 10.3 ). Astrocytes play important roles in constraining retinal vessels to the retina and in maintaining their integrity. At the arterio-venous crossing sites the deeper vessels may indent the retina as far as the outer plexiform layer. The retinal arterioles give rise to a plexus of capillaries (~5 µm in diameter). These capillaries form an interconnecting two-layer network. The first layer is located in the nerve fiber and ganglion cell layer and the second lies deeper, in the inner nuclear layer. In the peripapillary area an additional capillary network lies in the superficial portion of the nerve fiber layer, constituting the radial peripapillary capillaries distributed around the optic disk and along the temporal superior and inferior retinal vessels.

Towards the periphery, the deep capillary net disappears leaving a single layer of wide-mesh capillaries. Similar anastomotic capillaries connect the perifoveal terminal arterioles with the venules, leaving a capillary free zone of 400–500 µm in diameter. At the extreme retinal periphery an approximately 1.5 mm wide area is avascular. A capillary free zone also surrounds the arterioles, probably resulting from the local high oxygen tension affecting the capillary remodelling during maturation of the retinal vascular system.
The terminal vessels, namely the precapillary arterioles and the postcapillary venules, are linked through the capillary bed, the venous system presenting a similar arrangement as the arterioles’ distribution. The central retinal vein (CRV) leaves the eye through the optic nerve to drain venous blood into the cavernous sinus.
Vascular supply of the choroid
The vasculature of the choroid derives from the OA via branches of the 2–3 nasal and temporal main CAs and the ACAs, which supply the corresponding hemisphere of the choroid. , The main CAs branch into 10–20 short PCAs, which enter into the globe at the posterior pole and assume a paraoptic and perimacular pattern before branching peripherally in a wheel-shaped arrangement, and two long PCAs. Secondary and tertiary branches of the short PCAs are subsequently divided into the major choroidal arteries. , Some branches of these PCAs are selectively directed to the macular region vessels, namely the very short CAs.
Paraoptic pattern
Medial and lateral paraoptic short PCAs converge towards the ONH and form an elliptical anastomotic circle, the so-called circle of Haller and Zinn, through the formation of superior and inferior perioptic optic nerve arteriolar anastomoses ( Fig. 10.4 ) The circle of Haller and Zinn is incomplete in 23 percent of cases and complete with narrowed sections in 33 percent of cases. These variations could possibly make this part of the optic nerve head (ONH) circulation particularly vulnerable to ischemic attacks. Paraoptic short PCAs also supply the peripapillary choroid, whereas distal short PCAs supply only the peripheral choroid.

Perimacular pattern
The PCAs follow long, oblique intrascleral courses and travel in the virtual suprachoroidal space, giving off recurrent branches to the macula and to the anterior choroid at the ora serrata. Recurrent branches supply choroidal areas at 3 and 9 o’clock meridians. The ACAs perforate the sclera at the insertion of the tendons of the muscles and pass through the suprachoroidal space to enter the ciliary body. They primarily supply the ciliary body and iris; in addition, the ACAs are divided into recurrent branches that supply the anterior choroid. The choroid is composed of the choriocapillaris layer, the medium vessels layer (Sattler’s layer) and the outer layer of large vessels (Haller’s layer). In primates, the medium layer contains large arteries measuring 40–90 µm, large veins measuring 20–100 µm and nerves. The choriocapillaris and the medium-sized choroidal vessels lie between the apical retinal pigment epithelium (RPE) of neuroepithelial origin and the outer choroidal pigment of neural crest origin. Two collagenous structures, one on the inside (Bruch’s membrane) and one on the outside (subcapillary fibrous tissue), are connected by the intercapillary pillars. Most medium-sized vessels are external to the subcapillary fibrous tissue. Some branches of the short PCAs are selectively directed to the macular region. They have a spiral-shaped configuration, consistent with the vascular pattern of the arterial phase in indocyanine angiography. This pattern differs from that of those short PCAs which are not directed to the macular area, in that it expands in a typical chevron (V-shaped) configuration ( Fig. 10.5A ).

In vivo studies show that the PCAs and all their branches right down to their terminal arterioles and the choriocapillaries have segmental distribution with no functional anastomoses between them and thus behave as end-arteries. , The borderline area between the territories of distribution of any two end-arteries is defined as watershed zone, considered as an area of comparatively poor blood flow supply, more vulnerable to hypoxia–ischemia. In exudative age-related macular degeneration (AMD), a stellate pattern of the watershed zone is often observed. Choroidal neovascularization arises within this watershed zone in 88 percent of AMD patients. The relation between the site of choroidal neovascularization and the macular watershed zones suggests that these zones may be vulnerable to choroidal neovascularization induced by hypoxia–ischemia in AMD.
The choriocapillaris are distributed as a dense network of one layer of freely connected capillaries in the peripapillary and submacular area. This pattern changes to a lobule-like arrangement in the posterior pole and to a palm-like organization more peripherally. Each lobule, 0.6–1.0 mm diameter, consists of a capillary meshwork with radial and circumferential arrangement with an arteriole in the middle and a venule at its periphery ( Fig. 10.5B ). , Lobules in the equatorial part of the choriocapillaris are larger (200 µm) than those located both at the posterior pole (100 µm), and in the submacular area (30–50 µm). The lobules subdivide the choroid into several functional islands. The choroidal capillaries are connected by a discontinuous row of “zonulae occludens”. The part of the choriocapillaris that faces the RPE has large fenestrations, larger and more numerous in the submacular area, covered by a thin membrane with a central thickening. , Blood is discharged from the lobules of the choriocapillaris by collecting venules that join the afferent veins. At the posterior pole, a venule is located at the periphery of the lobule, stays on the same plane of the lobule, and possibly also drains adjacent lobules. Intervenular channels between choriocapillaries collecting venules and larger veins, direct arteriovenous anastomosis, and interdigitation between the choriocapillaris and venules are present in humans. , The meshwork of the venous plexus becomes less dense with increasing distance from the macula and the vessels become straighter, losing the tortuous aspect, which is characteristic of the macular region ( Fig. 10.5C,D ). Vessels of larger lumen form the subcapillaris plexus and eventually flow into the vortex veins. Four to six vortex veins are located 2.5–3 mm posterior to the equator, closer to the vertical meridian than to the horizontal one and drain into the superior and inferior orbital veins. Some drainage also occurs through the anterior ciliary veins of the ciliary body. Segmental venous drainage with watershed areas oriented horizontally through the disk and fovea and vertically through the papillomacular area is observed. ,
Fine structure and innervation of retinal and choroidal vessels
Retinal arteries differ from arteries of the same size in other organs in that they have an unusually well-developed smooth muscle layer and lack an internal elastic lamina ( Fig. 10.6 ). The smooth muscle cells are oriented both circularly and longitudinally, each being surrounded by a basal lamina that contains an increasing amount of collagen toward the adventitia. Retinal arteriolar precapillary annuli are observed in a number of animals at the arterial side-arm branches, but not in humans.

The capillary wall is composed of three distinct elements: endothelial cells, intramural pericytes, and a basement lamina. At their thickest point the endothelial cells present a nucleus bulging into the vessel lumen and express several cytoplasmic processes. Tight junctional complexes are found along the opposing surfaces of adjacent cells. The continuous endothelial cell layer is surrounded by a basal lamina within which there is a discontinuous layer of intramural pericytes in almost a one-to-one ratio with the endothelial cells. The recognition that pericytes are highly contractile cells, coupled with their uniquely high representation in the retinal microvasculature has led to the hypothesis that these cells play an important role in the regulation of retinal blood flow. However, direct in vivo evidence for this role is still lacking.
Histologic studies and autonomic nerve stimulation experiments reveal a rich supply of autonomic vasoactive nerves to the choroid but not to retinal vessels. Sympathetic nerves derived from the superior cervical sympathetic ganglion innervate the choroidal vascular bed as well as the CRA up to the lamina cribrosa but not further into the retina. , Nevertheless, along the ONH and within the retina, α- and β-adrenergic receptors and receptors for angiotensin II are present. As sympathetic nerve fibers to other tissues, the choroidal sympathetic nerve fibers are immunoreactive to neuropeptide Y.
In the human choroid there are not only numerous nerve fibers but also choroidal ganglion cells (CGCs). , Histochemical and immunohistochemical studies performed in whole mount preparations allowed precise classification and quantification of these CGCs. A comparative study of different mammalian eyes demonstrated that larger numbers of CGCs (up to 2000/eye in humans) are present only in eyes of primates with a well-developed fovea centralis, namely humans and higher monkeys. These CGCs stain for neural nitric oxide (NO) synthase (nNOS) and vasoactive intestinal polypeptide (VIP). , Targets innervated by these CGCs include arteries and non-vascular smooth muscle cells attached to the choroidal elastic network and the walls of the choroidal arteries. Thus, these CGCs seem to be involved in control of choroidal thickness and blood flow. In addition, the postganglionic nerve fibers of the CGCs presumably support the vasodilative function of the facial nerve.
The parasympathetic system provides vasodilating fibers to the choroid through the facial nerve, as confirmed by stimulation experiments in rabbit, cat, and monkey. , VIP immunoreactive nerve fibers, originating in the pterygopalatine ganglion, have been observed around choroidal blood vessels in several species, including man. Furthermore, the parasympathetic perivascular nerves are immunoreactive for both nNOS and VIP. , Additional neuropeptides like peptide histidine isoleucine (PHI) and pituitary adenylate cyclase polypeptide (PACAP) could be present in some of the parasympathetic nerve fibers as well.
Vascular supply of the anterior segment
The vascularization of the anterior segment originates from the ACAs and the long PCAs. These PCAs have an intrascleral course beneath the medial and lateral rectus muscles. They run forward to the ciliary body and the iris, where they give rise to their terminal branches. The ACAs have an extraocular course within the rectus muscles. Close to muscle tendons, they exit the muscles and run radially towards the limbus within Tenon’s capsule. Close to the limbus they give rise to superficial (episcleral) and deep (scleral) branches. , The episcleral arteries run forwards in the episcleral space; near the limbus they assume a circumferential course and anastomose each other to form a fragmentary episcleral arterial circle ( Fig. 10.7 ).

Penetrating vessels through the sclera give rise to branches that vascularize the iris (major arterial circle of the iris) and the ciliary body (intramuscular arterial circle). In addition, species-dependent intramuscular arterial circle located within the ciliary body has been described. The penetrating vessels give rise, through recurrent branches, to a communication between the ACAs and PCAs.
The vascular supply of the ciliary processes is complex and species-related. Three territories of supply have been described in humans. The first territory, situated mainly at the broadened base of the anterior edge of the major ciliary process, is irrigated by anterior arterioles leading to a capillary network. The capillaries drain into venules located deep in the ciliary processes with little connection to the other vascular territories. The second territory is also derived from the anterior portion of the major ciliary processes and drains into a broad marginal vein at the inner edge of the ciliary process. The third territory provides blood irrigation to the posterior of the major ciliary processes and the minor ciliary ones. A schematic representation of the vascular supply of the human ciliary body is shown in Figure 11.2B .
The arterial supply of the iris originates mainly from the long PCAs and the ACAs. The long PCAs divide themselves in terminal branches to form the big arterial circle of the iris circumscribing the pupil. This circle receives also the contribution of the ACAs. Thus, the iris is supplied by the posterior and anterior ciliary net. , Vessels with radial trajectory and a corkscrew arrangement to take care of pupillary movement arise from the iris big arterial circle to reach the rim of the pupil. They divide to form the minor arterial circle of the iris. The capillaries within the iris are difficult to see; they are localized mainly in the pars pupillaris. The venules originate from the pars pupillaris of the iris and run in parallel, but in more depth than the arteries to the root of the iris, increasing their diameter. At this level, they receive also a contribution from the ciliary body and head for the suprachoroidal space before reaching the vortex veins.
Transport through blood–retinal barriers
Optimal cell function requires an appropriate, tightly regulated environment. This regulation is determined by cellular barriers, which separate functional compartments, maintain their homeostasis, and control transport between them. The close inter-relationship of epithelia and vascular endothelium with extracellular structures, namely extracellular matrix and glycocalyx, may modulate the dynamic responsiveness of barrier cells. As reviewed elsewhere, two major pathways control the passage through barriers, namely the transcellular pathway involving vesicles, specific carriers, pumps, and channels and the paracellular pathway through the intercellular cleft.
Transcellular pathway (transcytosis)
The transcellular pathway actively and passively transports water, ions, non-electrolytes, small nutrients, and macromolecules in an energy-dependent manner. Most proteins are transported non-selectively within vesicles, either in their fluid phase or adsorbed at the vesicular membrane (vesiculo-vacuolar transport). Constitutive transcytosis (the process by which macromolecules are transported across the interior of a cell) of albumin across the vascular endothelium is particular in that each transcytotic routing, whether receptor-mediated, by adsorption or as bulk fluid, is implicated in regulating the transvascular oncotic pressure gradient of albumin. , Fenestrations of the endothelial cell membrane are characterized by high permeability. They are found in choroid plexi of the brain, in the choroid and in the neovessels formed during the angiogenic processes ( Fig. 10.8 ).

Paracellular pathway
Tight junctions confer firm intercellular adhesion and regulate paracellular permeability through the intercellular cleft. Indeed, barrier properties depend on the specific molecular architecture of the tight junctions. Tight junctions are polymeric adhesion complexes with a transmembrane component composed of occludin ( Fig. 10.9 ), claudins and junction adhesion molecules, linked to a cytoplasmic plaque containing, among other components, zonula occludens protein and cingulin.

The plaque itself is anchored to the actin cytoskeleton and to various signaling molecules that participate in the control of cell proliferation and differentiation. In general, water, ions and small non-charged solutes employ the paracellular pathway by passive diffusion and with low selectivity along electrochemical and osmotic gradients that are built up by the activity of transcellular transporters or, by external gradients of solutes. The characteristics of both paracellular and transcellular pathways determine the preference for small solutes for either pathway and, therefore, are important parameters for drug absorption. Drugs with high lipid solubility readily cross the lipid cell membrane bilayer, but could still be poorly absorbed into the eye due to efflux pumps in the ocular barriers (see Chapter 17 ).
Extracellular structures
Glycocalyx
The apical and luminal surfaces of epithelial and endothelial cells are endowed with macromolecules that are bound to the plasma membrane and build up the glycocalyx. This coat, composed of glycoproteins, proteoglycans, and glycosaminoglycans, is polyanionic and thus negatively charged. Molecules like fibrinogen and albumin, enzymes, cytokines and growth factors, associate with the glycocalyx thanks to their cationic charge. By these particular electrostatic features, the glycocalyx acts as a charge-selective barrier that determines the accessibility of certain molecules to the plasma membrane.
A major function of the glycocalyx of the vascular endothelium is to sense mechanical forces generated by the blood flow and modulate the shear stress-induced release of NO. Long-standing degradation of distinct components of the glycocalyx contributes to increased vulnerability of the endothelial cells, barrier function, block mechano-transduction and lead to inhibition of NO production that eventually abolishes vasodilation.
Extracellular matrix
This matrix (ECM) consists of higher-order assemblies of numerous components that are secreted in a cell-type-specific manner. , The major barrier tissues, epithelium and vascular endothelium, both synthesize a specialized ECM scaffold, the basal lamina, at their basal and albuminal side, respectively. Basal laminas serve as cell attachment sites by means of a variety of transmembrane receptors that are able to transmit signals between cell and ECM in either direction. The best known among these receptors are the integrins, the specificity of which is determined by the molecular characteristics of the underlying ECM. Disruption of integrin-matrix binding causes the detachment of the cells from their substrate and also causes an increase in the permeability of tight junctions. , The interactions of cell to cell-matrix are of great importance to insure intercellular permeability in microvascular systems. Physical, oxidative stress, and inflammatory events may affect the permeability of the intercellular junctions. ,
Inner blood–retinal barrier
The endothelium of intraretinal blood vessels is considered as the main component of the inner blood–retinal barrier (BRB) resembling the blood–brain barrier in that both separate blood from neural parenchyma. The presence of a complex network of tight junctions, the absence of fenestrations and a relative paucity of caveolae make up the tightness of the iBRB. Numerous transport systems account for the selectivity of the barrier, such as the transport system for glucose across the retinal capillary endothelial cells of the inner BRB, which is mediated by the sodium-independent glucose transporter GLUT1.
Pericytes
Both pericytes and smooth muscle cells provide structural support to the vasculature. The increased density of pericytes in the retinal microvasculature as compared to other organs , has led to the presumption that pericytes play a role in the regulation of retinal perfusion by contraction and relaxation. , Circumstantial evidence for contractility of pericytes is the expression of a number of contractile proteins, in particular α-smooth muscle actin, desmin, and non-muscle myosin. Moreover, pericytes express receptors for vasoactive substances, enabling them to respond by contraction or relaxation. Recent work suggests that pericytes, rather than endothelial cells, control capillary diameter, because diameter changes do not occur in pericyte-free regions. Thus, pericytes would play a major role in redirecting blood flow at the capillary level. However, direct in vivo evidence for this role in the retina is still lacking.
Pericytes modulate barrier permeability since they express a high number of caveolae that appear to be involved in transcellular transport. Moreover, pericytes and smooth muscle cells participate in the permeability properties of the basal lamina by secretion of matrix components. Deficient contractility of pericytes and smooth muscle cells may thus have a negative impact on the regulation of blood flow that, in turn, may impair pericyte–endothelial interactions, critical for the maintenance of the BRB.
Glial cells
In the retina, both astrocytes ( Fig. 10.3 ) and Müller cells participate in vessel ensheathment, whereby astrocytes are confined to the optic nerve fiber and the ganglion cell layer. Glial cells play an important role in vessel integrity and barrier properties, both via direct contact and through release of humoral factors. Glial cell line-derived neurotrophic factor (GDNF) has been recognized to enhance barrier tightness, whereas transforming growth factor-β (TGF-β) appears to decrease it. Several other growth factors and cytokines, such as tumor necrosis factor-α (TNF-α), interleukin-6 (IL-6), and vascular endothelial growth factor (VEGF) are produced by retinal glial cells and affect the tightness of the iBRB. VEGF directly downregulates tight junctional proteins, evoking a decrease in transendothelial resistance ( Box 10.1 ).
VEGF is the predominant vasoproliferative factor involved in the development of retinal or subretinal neovascularization, occurring either during the evolution of retinal ischemic microangiopathies, or exudative neovascular AMD. Ocular neovascularization regresses following intravitreal injections of monoclonal antibodies inhibiting isoforms of VEGF-A. Anti-VEGF treatment is actually the predominant approach for the treatment of neovascular AMD.
Outer blood–retinal barrier
The outer blood–retina barrier (BRB) is composed of three structural entities, the fenestrated endothelium of the choriocapillaris, Bruch’s membrane, and the retinal pigment epithelium (RPE).
Fenestrated endothelium
The fenestrations of the choriocapillaris are covered by a thin membrane with a central thickening. , They have high permeability, similar to that of an isoporous membrane with a pore diameter of 4 Å, which probably accounts for the maintenance of an adequate concentration of glucose and other nutrients at the RPE level.
Retinal pigment epithelium
The RPE cells are equipped with an elaborate transcellular pathway system and an apico-lateral seal formed by intercellular junctions, the adherens junction and the tight and gap junctions, as well as, in some species, desmosomes. The RPE is considered as a relatively tight epithelium with a 10 times higher paracellular than transcellular resistance ( Fig. 10.10 ). ,

Nutrients and vitamin A are transported in basolateral to apical direction from the blood to the photoreceptors. In the opposite direction, transcellular transport from the subretinal space towards the choriocapillary bed is required for removal of metabolites, water, and ions. The transepithelial ion transport is linked to the transport of lactic acid, the major metabolic end product of neuronal function.
Bruch’s membrane
Using electron microscopy, five distinct layers of Bruch’s membrane have been defined: the two basal laminas of the RPE and the endothelium of the choriocapillaris, the inner and outer collagenous layers, and a central discontinuous elastic layer. , Bruch’s membrane provides tensile strength and, thanks to the presence of proteoglycans, constitutes a reservoir of growth factors. Its overall negative charge is due to the high content of glycoconjugates and it is responsible for charge-selective restriction to the passage of ions and solutes.
Blood–aqueous barriers
Secretion of the aqueous humor and its transport towards the posterior chamber is controlled by the blood–aqueous barrier (BAB). These barriers, which possess active transport mechanisms, include the endothelium of the iris capillaries, the iris posterior epithelium, and the non-pigmented posterior epithelium of the iris. The passive permeability of the BAB depends on the ionic concentration gradients.
Composed of iris capillaries and pigmentary epithelium, the anterior BAB allows the transcellular transport by means of vesicles. The paracellular transport is controlled by the extension of the tight junctions. Associated with the ciliary and retinal pigment epithelium, the iris pigment epithelium seems to constitute an obstacle to the passage of type T activated lymphocytes. The anterior surface of the iris, formed by only one layer of fibroblasts, does not constitute a barrier. It allows free access of the aqueous humor to the stroma and the iris muscles, thus causing the rapid resorption of drugs present in the aqueous humor.
The posterior BAB is formed by tight junctions, which are present on the lateral pole of the cells of the non-pigmented ciliary epithelium ( Fig. 10.11 ). These tight junctions are permeable to small, non-ionic molecules, such as sucrose. The capillary endothelium of the ciliary stroma possesses fenestrations that make its permeability relatively high. In contrast, the capillaries of the ciliary muscle appear relatively tight, similar to those of the iris.

Techniques for measuring ocular blood flow
Decades of technical developments have led to a number of methods to obtain quantitative information on ocular blood flow (BF) in animals and humans.
Techniques used in experimental animals
These techniques have been valuable for quantitative determinations of BF in experimental animals. They include direct measurements of BF through cannulation of uveal veins in rabbits and cats, and radioactively labeled microspheres. Calorimetry, or heated thermocouple, radioactive krypton desaturation, hydrogen clearance, iodoantipyrine I It should be I 125, 5, laser speckle phenomenon-/flowgraphy, targeted dye delivery, leukocyte dynamics by fluography, and invasive laser Doppler flowmetry have been used to determine changes in flow in the various ocular tissues.
Intracardiac injections of radioactively labeled, non-labeled colored or fluorescent microspheres permit determination of regional BF to the different tissue beds within the eye by analyzing the distribution of the microspheres. Ideally, the relative quantity of particles recovered in each organ, compared to the total number of injected particles, equals the fraction of the cardiac output to each organ. The precision of the various microsphere techniques depends on several factors, including the number of particles trapped in the tissue sample. The accuracy of BF measurements within small tissue samples, such as the retina, the iris, or the optic nerve, can be improved if the number of samples is increased.
With direct cannulation of the uveal veins, the BF within the uveal tract has been measured in rabbits and cats. In cats, there is a large intrascleral venous plexus that permits sampling of venous blood from either the anterior uvea or the posterior choroid. This plexus has been used to determine arteriovenous differences of oxygen and glucose. A similar plexus exists in the retrobular space of the rabbit eye. Retinal arteriovenous differences can be studied in a variety of animals, including the pig, in which the retinal veins form a ring-shaped plexus around the optic nerve within the retrobulbar space.
Studies of the oxygen partial pressure profile within the retina with microelectrodes have provided information on the relative importance of the choroidal and retinal circulation in providing oxygen to the retina. , Glucose consumption in vivo can be studied by determining the tissue uptake of labeled 2-deoxy- d -glucose. Although it is not a direct measure of BF, this technique gives an estimate of alterations in metabolic demands and has proved very useful for studying the effects of increased intraocular pressure (IOP) and light on the retina and the optic nerve. ,
Non-invasive techniques used in physiological and clinical research
A number of techniques have been developed to obtain quantitative information on the physiology, pharmacology, and pathology of the blood circulation in the different ocular vascular beds.
The diameter (D) of retinal vessels has been measured in the past from magnified fundus photographs using a caliper or by scanning across the vessels. In recent years, the Retinal Vessel Analyzer (RVA) has simplified markedly this measurement, allowing also the quasi-continuous recording of D changes evoked by various physiological maneuvers (dynamic measurements) ( Fig. 10.12 ).

The mean circulation or transit time (MCT or MTT) of fluorescein injected intravenously through a retinal segment ( Fig. 10.13A ) has been assessed from digitized fluorescein angiograms. The gray scale level is used as an estimate of the relative dye concentration (dilution curves) in the artery feeding and the vein draining the segment and the MCT / MTT has been determined from these dilution curves ( Fig. 10.13B ). Specific conditions related to the retinal segment, the properties of the dye, the mode of its injection, the method of recording the dilution curves and the correction for dye recirculation must be fulfilled to obtain precise data on MCT / MTT. In the application of this technique to the eye, some of these conditions cannot be strictly satisfied, particularly in cases of retinal vascular pathology (for instance, in proliferative retinopathy, diffusion of fluorescein from the retinal vessels may distort the dilution curves), so that the measured MCT / MTT must be regarded as an approximation of the true MCT / MTT. Other parameters have been derived from the time course of the fluorescence intensity: the mean velocity of the dye (MVD) in a retinal artery, the arterio-venous passage time (AVP), which is the time difference between the first appearance of the dye at a reference point at the temporal retinal artery and that at the adjacent vein. Contrary to the MCT, which gives more weight to the peripheral retinal circulation, the AVP represents mainly the passage time of dye through the shortest segments close to the papilla and thus does not reflect the flow of fluorescein through the periphery of the retina. A study in monkeys, undertaken to determine the correlations between MCT and AVP and retinal BF, the latter measured by labeled microspheres, showed that these correlations were not statistically significant (p > 0.05), even in the case of calculation of the MCT using the more sophisticated impulse response technique. Clearly, MCT and AVP data should not be interpreted in terms of BF.

For studies on the choroidal circulation, indocyanine green (ICG) is a more suitable dye. ICG has two significant advantages over fluorescein for choroidal angiography: the fluorescence of ICG is in the near infrared and, therefore, not blocked by the pigment epithelium and ICG is almost completely bound to proteins, which means that it does not easily pass the walls of the choriocapillaris. Single choroidal vessels can be observed even late in the angiogram. The need for a special camera and frequent difficulty in interpretation of ICG angiograms have somewhat limited widespread acceptance. An obvious use for ICG is to examine subretinal neovascular membranes, where it is a valuable complement to, but not a substitute for, fluorescein angiography. Using recently developed techniques allowing simultaneous optical coherence tomography and ICG fluorescence may offer a new approach to clinical studies of choroidal blood flow.
Bidirectional laser Doppler velocimetry (BLDV) allows the measurement of absolute blood velocity. BLDV is based on the Doppler effect ( Fig. 10.14 ). Retinal BF (in µl/min) in the main retinal vessels is calculated from the centerline velocity ( V max ) of the red blood cells (RBCs) combined with D measurements of these vessels and calculating the instantaneous
B F m e a n = π D 2 4 V m e a n

V mean represents an average over the vessel cross-section. The average RBC velocity during the heart cycle is obtained by integrating V mean over this cycle. For a parabolic velocity profile, V mean = V max /2. The assumption of this type of profile is justified when measurements are performed at straight portions of a vessel, a few vessel diameters away from an arterial branching or a venous junction ( Fig. 10.15 ), as recently confirmed by Doppler Optical Coherence Tomography. , In the human and primate monkey eyes, the velocity in the main retinal arteries shows a strong pulsatile component and V max increases linearly with D, as found in most vascular beds. ,

where V(d) represents the velocity at a distance d from the internal wall. d o is the position of the center of the vessel. R i is the internal radius of the vessel. V max is the centerline (maximum) velocity. K = 2.38 in the systolic phase and 1.94 in the diastolic phase.
Laser Doppler flowmetry (LDF) allows the measurement of the change in flux of RBCs in the superficial layer of the ONH, the subfoveal choroid, and the iris. Calculation of this flux is based on the model of Bonner and Nossal ( Fig. 10.16A ). The change in flux is proportional to the change in BF if this change is not accompanied by a change in hematocrit. LDF can operate in a dynamic or scanning mode. The dynamic mode, which records the flux in a continuous way at discrete sites of the capillary bed, is particularly appropriate for recording changes of flux over time. The scanning mode provides a two-dimensional image of the flux in the capillaries of the optic disk and peri-papillary retina, as well as an intensity image of the retinal vessels perfused ( Fig. 10.16B ).

The temporal variations of laser speckle resulting from the interference of laser waves scattered by the tissue can be used to determine the velocity of the RBCs in the ONH, retinal and choroidal circulations. , This laser speckle flowgraphy and the LDF approaches are different ways of looking at the same phenomenon. Both techniques measure at a single point in the tissue. In both cases, adding scanning provides a map of the spatial velocity and flux. , One important point when applying these laser-based techniques is that the measured flux depends on the scattering and optical absorption properties of the tissue. Therefore, direct comparison between flux values from different eyes may not be valid due to variations in the scattering properties resulting from differences in tissue structure and composition. Similarly for a valid comparison between flux values obtained at different times in the same eye by dynamic and scanning LDF and laser speckle flowgraphy, the tissue must be assumed to maintain the same scattering properties over time.
The velocity, number and velocity pulsatility of leukocytes moving in the retinal capillaries of the macular region has been quantified using the blue field simulation technique, which is based on the entoptic perception of one’s own leukocytes. Visual acuity must be better than 20/50 for a reliable measurement. The ability of a subject to do the blue field simulation test can be assessed by having subjects match the speed and number of two simulated leukocyte’s motions displayed on computer screens. Blue field data have been confirmed by the objective SLO-adaptive optics imaging technique.
Color Doppler imaging combines B-scan imaging of tissue structure, color representation of blood velocity based on ultrasound Doppler-shifted frequencies, and pulse-Doppler measurements. , At the level of the OA, CRA, and PCAs, the maximum and minimum values of the velocity are identified to determine the peak systolic (PSV) and end diastolic (EDV) values of this velocity from which a resistivity index (RI) is obtained:
R I = ( P S V − E D V ) P S V
RI is often considered as a measure of the downstream vascular resistance. However, experimental data show that RI in CRA is not a satisfactory measure of this resistance. Studies on cerebral vessels have not confirmed a useful correlation between RI and cerebrovascular resistance.
Pneumatonometric measurement of ocular pulse amplitude estimates the pulsatile component of choroidal BF (POBF). , Since the ratio of pulsatile/non-pulsatile ocular BF cannot be assumed to be constant, especially in the event of changes in systemic blood pressure, pulsatile BF is not a good estimate of total ocular BF. Another technique that makes use of the pulsatile nature of the IOP is the interferometric measurement of the IOP-induced variation of the distance between cornea and retina to estimate changes in choroidal blood volume during the cardiac cycle.
The information given here on ocular BF techniques is far from exhaustive. Additional details can be found in a number of excellent reviews.
Ocular circulatory physiology
General hemodynamic considerations
BF through a blood vessel depends upon the perfusion pressure (PP), the pressure that drives blood through the vessel, and the resistance (R) generated by the vessels. For an incompressible uniform viscous liquid (dynamic viscosity η) flowing through a cylindrical tube (length L) with radius (r), BF is given by the Hagen–Poiseuille law: BF = PP/R, where R = ηL/2πr 4 . Many factors make it difficult to directly apply this law to a microvascular bed. These include the η-dependence on local hematocrit, the changes in the velocity profile of the RBCs and shear rate at branchings and junctions and others. Another approach at characterizing BF through a system of vessel is based on Murray’s law, which says that through each vessel of a circulatory system with optimal design (blood flowing with minimal loss of energy) BF = k(r 3 /√η). The constant k depends upon the lengths and the radius of the vessels.
The mean ocular PP driving blood through the eye is the mean blood pressure in the ophthalmic artery (OA) minus the pressure in the veins leaving the eye. The venous pressure is close to the IOP. With the subject in the sitting or standing position, mean ocular PP is about 2/3 of the mean brachial artery blood pressure (ABP), i.e.
2 / 3 [ A B P d i a s t + 1 / 3 ( A B P s y s t − A B P d i a s t ) ] − I O P
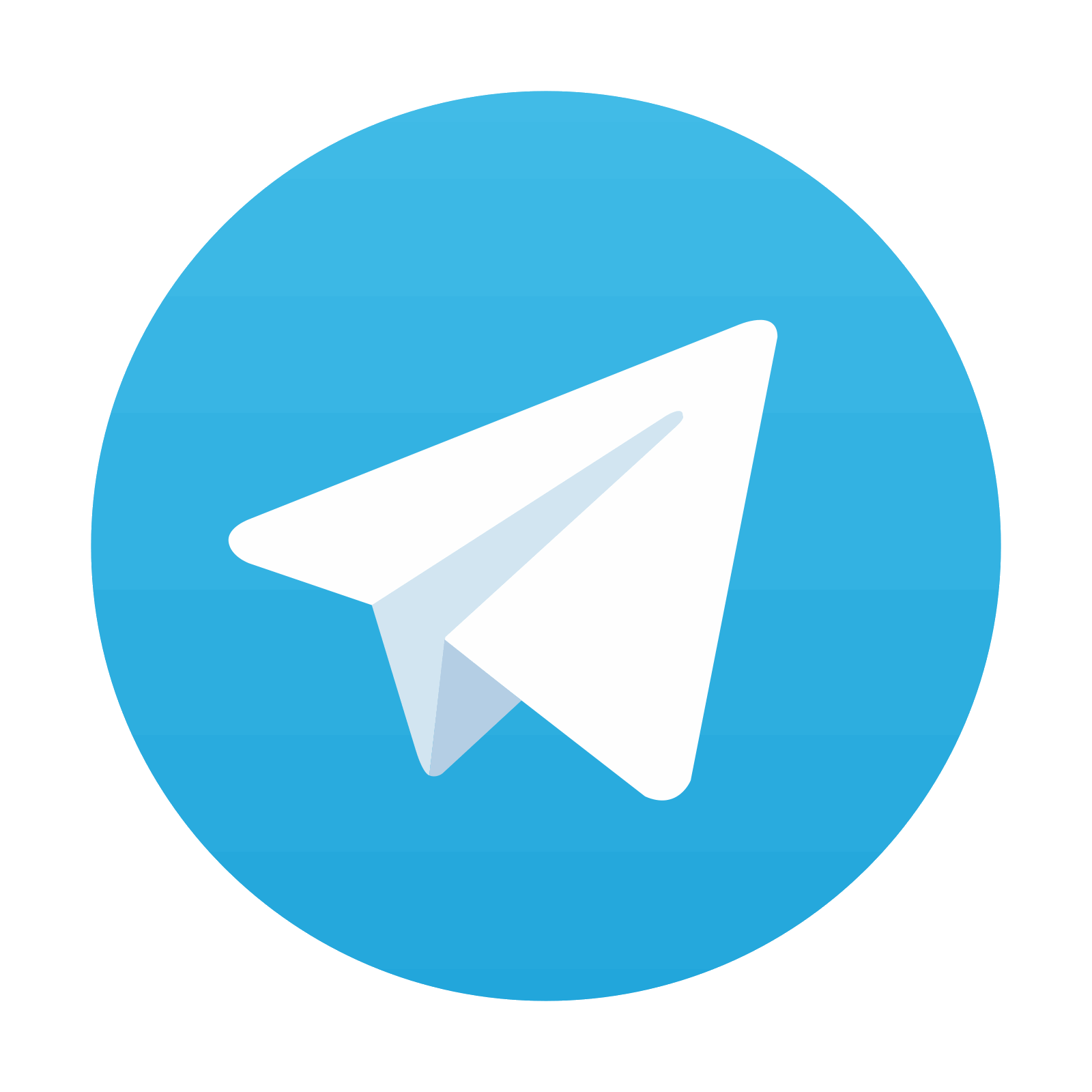
Stay updated, free articles. Join our Telegram channel
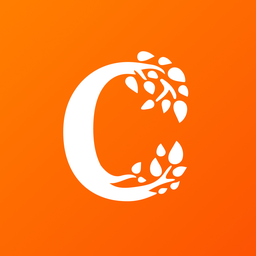
Full access? Get Clinical Tree
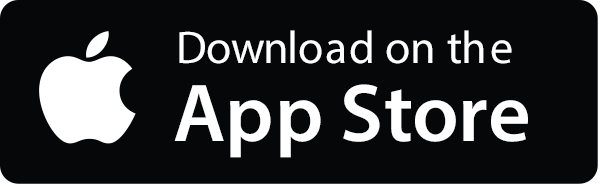
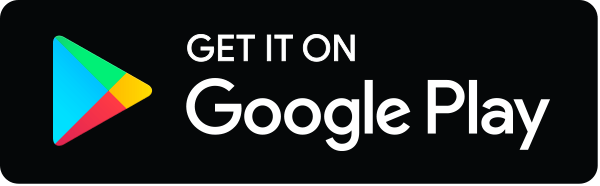