Novel Radiation Therapy Techniques in the Management of Head and Neck Cancer
Avraham Eisbruch
In traditional irradiation of head and neck cancer, the placement of the radiation fields and their shapes are based on the bony anatomy acquired by the simulator diagnostic-quality films. During the late 1980s, advancements in computer technology and imaging introduced methods to identify the targets on computed tomographic (CT) scans and display the radiation beams in three dimensions relative to the anatomy. In addition, calculation and display of the radiation dose distributions and methods to evaluate and compare rival plans using dose-volume histograms (DVHs) became available. The introduction of multileaf collimators (MLCs) facilitated an increase in the number of beams that could be delivered without a large extension of treatment time. Treatment could now be delivered from multiple angles, including noncoplanar directions, when required. The result was the emergence of three-dimensional conformal radiotherapy (3DCRT), which allowed better precision of irradiation delivery to image-based targets, and some improvements in the sparing of uninvolved critical organs. Early studies of the utility of 3DCRT in head and neck cancer examined cancer of the larynx,1 naso-pharynx,2,3 hypopharynx,4 and paranasal sinuses.5 These studies demonstrated a significant benefit from 3DCRT such as better coverage of the tumors and reduced doses to critical tissue compared with standard techniques. A step forward has been gained in the mid-1990s by intensity-modulated radiotherapy (IMRT), which facilitated a higher degree of dose conformality and offered opportunities for additional clinical gains. More recently, imaging-guided radiotherapy (IGRT) has emerged, providing opportunities for better precision of patient setup and for tracking tumor regression and anatomic changes in the noninvolved tissue during the course of radiation. Tracking these anatomic changes may facilitate future replanning during the course of therapy to address them, a concept referred to as adaptive radiation therapy (ART). Other emerging technologies include new imaging modalities that provide opportunities to study the customization of the radiation dose depending on the expected tumor biology, and the use of heavy particles like protons or carbon ions, which offer additional advantages in dose conformality. In addition, technical innovations in the delivery of IMRT, such as tomotherapy and volumetric-modulated arc therapy (VMAT), provide specific improvements and introduce specific trade-offs compared with beamlet-based IMRT. Some recent experience has been gained with stereotactic radiotherapy using a robotic radiosurgery system for recurrent cancers near the base of the skull. These advancements will be described in this chapter, emphasizing the role of IMRT, for which ample clinical experience has accumulated.
IMRT: GENERAL ASPECTS
IMRT implies the use of radiation fields for which intensity varies across the field, depending on the thickness of the target and the existence of critical organs or critical noninvolved tissue in their path. Treating the targets with multiple beams of varying intensity allows a relatively uniform dose in an irregularly shaped target while avoiding a high dose to the surrounding structures. Two technologic developments made IMRT possible: the introduction of computer-controlled MLCs and the development of computerized optimization or “inverse planning,” which determines the intensity of the beams required to satisfy a specified set of dose distributions.
Currently, most IMRT delivery systems use conventional MLCs. Delivering IMRT using a conventional MLC can be made in a “dynamic” or “static” form. In the “dynamic” form, the leaves at each gantry position are swept across the target while the beam is on and their speed determines the radiation fluence. In “static” or “segmental” multileaf IMRT, each field consists of multiple segments with different intensities. These forms of IMRT are currently offered by most manufacturers of linear accelerators. A helical tomotherapy design has been developed at the University of Wisconsin and is similar in its operation to the helical CT, delivering essentially an infinite number of beams while the gantry rotates around the patient. This design has been available commercially in recent years.
The complexity of IMRT plans cannot usually be achieved by the forward, iterative process alone used in 3DCRT plans. Instead a computer-based optimization process, called inverse planning, is used. Inverse planning demands that the physician and planning team state the treatment goals, usually in terms of the dose and/or dose-volume goals for the targets, and the constraints for the critical normal tissue (the objective, or cost function). The computer attempts to achieve the desired outcome by iteration through numerous possible beam intensities. The desired solution is the dose distribution that minimizes the variance of the delivered dose relative to the objective function. For each target and noninvolved organ or tissue, an importance factor or weight is assigned, and the objective function is the sum of the variance terms representing each structure of interest, multiplied by the importance factor. Because the variance is the sum of the squares of the differences between the prescribed and the delivered doses, this approach is called a quadratic objective function and is the one used in most current optimization systems. A publication by the Intensity Modulated Radiation Therapy Collaborative Working
Group summarized the consensus regarding IMRT planning, delivery, commissioning, radiation safety and quality assurance, and detailed recommendations about target volume and dose specification.6 This publication is recommended for readers interested in more detailed discussions about the general aspects of IMRT.
Group summarized the consensus regarding IMRT planning, delivery, commissioning, radiation safety and quality assurance, and detailed recommendations about target volume and dose specification.6 This publication is recommended for readers interested in more detailed discussions about the general aspects of IMRT.
IMRT FOR HEAD AND NECK CANCER
The anatomy of the neck is complex, with many critical and radiation-sensitive organs in close proximity to the targets. Tight dose gradients around the targets that limit the doses to the noninvolved tissue, features characteristic of IMRT, are desirable and offer the potential for therapeutic gains. The sparing of uninvolved normal organs can offer significant improvement in outcomes. This includes major salivary glands, minor salivary glands dispersed within the oral cavity, the mandible, and the pharyngeal musculature as well as other organs pertinent to specific anatomic tumor locations. In the cases of nasopharyngeal and paranasal sinus cancer, critical normal tissue that may be partly spared using IMRT include the inner and middle ears, the temporomandibular joints, temporal brain lobes, and the optic pathways.
In addition to noninvolved tissue sparing, IMRT offers a potential for improved tumor control by reducing the constraints on the tumor dose due to critical organs, for example, the spinal cord, brain stem, and the optic pathways, which occasionally limit the tumor boost doses in conventional radiation therapy (RT). This is achieved by specifying a maximal dose to the critical organs and a high penalty in the optimization process if that dose is exceeded. In addition, IMRT eliminates the need for posterior neck electron fields, commonly used in conventional RT, and their associated dose deficiencies. IMRT in the head and neck is more feasible than in other sites as breathing-related organ motion is practically absent. However, there are other factors that need to be taken into account such as patient setup uncertainties, swallowing-related organ movements, and changes in target and organ positions related to tumor shrinkage and patient weight loss during therapy, detailed in the subsequent text.
Patient Selection
Head and neck IMRT is work intensive and lengthens treatment time. Not every patient is expected to benefit; those who would benefit most are patients with paranasal sinus cancer where the targets are near the optic pathways, patients with oropharyngeal or nasopharyngeal cancer in whom standard RT fields would encompass most of the salivary glands, and patients in whom standard techniques would require a compromise in the tumor dose, due to proximity of the tumor to the spinal cord or the brain stem. Patients with laryngeal cancer and clinically noninvolved neck, receiving treatment to the larynx alone or requiring irradiation of the neck encompassing the jugulodigastric nodes but not extending to the base of the skull, may not benefit from IMRT compared with simpler techniques. The same applies to patients requiring irradiation to the ipsilateral neck alone. In recent years, there have been suggestions to use IMRT when the glottic larynx alone is treated, to spare the carotid artery and reduce the risk of development of an atheromatous plaque and subsequent brain stroke.7,8 However, the utility and value of this uproach is not known and it is not practiced by most centers.
Immobilization
Head and neck immobilization is typically performed using a thermoplastic mask with several attachment points to the treatment table and a head support. Several commercial systems are available. Typically, immobilization with these systems results in average daily setup errors of few millimeters.9 These errors require an extension of the targets to ensure adequate irradiation (see “The Planning Tumor Volumes” in the subsequent text). It is important to extend the mask to include the lower neck and shoulders, if targets in the lower neck and the supraclavicular nodes are included in the IMRT plans. An alternative is to treat the lower neck with an anterior field and match this field with the upper neck IMRT fields using a split-beam technique. This strategy reduces the doses to the glottic larynx and to the skin of the lower neck and reduces treatment complexity, compared with whole-neck RT.10 Such a strategy is recommended in cases where the risk of disease in the lower neck is low. In these cases, the head and upper neck alone need to be immobilized.
Defining the Target: Imaging
In most cases, the simulation contrast-enhanced CT is in most cases the only imaging modality required for the delineation of the targets. Magnetic resonance imaging (MRI) is limited by its sensitivity to artifacts, difficulty in interpretation, long examination time, and cost. MRI is a necessary adjunct to CT for tumors close to the base of the skull, such as nasopharyngeal and paranasal sinus cancer. In these situations, MRI provides better details of tumor extension and the parapharyngeal and retropharyngeal spaces, compared with CT.11 Fluorodeoxyglucose-positron emission tomography (FDG-PET) has recently been found to add significantly to the information gained from CT regarding the tumor extent in lung cancer.12 In contrast, early series of head and neck cancer where CT, MRI, and FDG-PET were obtained, and surgery was then performed to validate the primary tumor extent and lymph node involvement, reported a rather limited benefit of FDG-PET compared with CT/MRI.13 More recent studies have better defined the role of FDG-PET as a tool for target delineation in IMRT of head and neck cancer. Daisne et al. performed pretreatment CT, MRI, and FDG-PET on 29 patients with stage II to IV laryngeal and oropharyngeal cancers.14 Nine of these patients subsequently underwent total laryngectomy, and these specimens were compared with coregistered radiographic images. There was no difference between total CT and magnetic resonance (MR) volumes, but GTVs obtained from FDG-PET were smaller than these two, and surgical specimens were even smaller, indicating overestimation of GTVs with all three imaging modalities. However, when examined in detail, despite overestimating in most dimensions, all three imaging modalities actually underestimated the mucosal extent of disease, highlighting the importance of the physical examination in determining the mucosal extent of tumors for treatment planning purposes. Another study, from Emory University, did not have pathologic correlation, but imaged 40 patients with FDG-PET and CT.15 In this report, the target according to PET was smaller than the CT-defined target in 75% of cases, but larger in 18%. When the PET images were registered on the treatment plan constructed with only CT volumes, the PET-defined target was noted to be underdosed in 25% of cases. It is possible that the difference in the results of these two studies can be attributed to different thresholds for determining PET positivity. Whereas fixed thresholds have been commonly used, the group from the Universite Catholique de Louvain in Brussels, Belgium, has published work suggesting that the correct threshold depends on the signal-to-background ratio of each study and is typically between 36% and 73%.16 The practice at the University of Michigan has been to combine the information gained from FDG-PET and CT (and MRI for tumors near the base of the skull) for a composite gross target, as the information gained from any single imaging modality cannot be considered gold-standard taking into account current published studies. In all cases, the findings of a careful clinical examination
form the basis for assessing the extent of the primary tumor. For example, palpation of oropharyngeal tumors can assess their extent in a way that is complementary to imaging, especially the mucosal extent which is poorly assessed by imaging.14
form the basis for assessing the extent of the primary tumor. For example, palpation of oropharyngeal tumors can assess their extent in a way that is complementary to imaging, especially the mucosal extent which is poorly assessed by imaging.14
Selection and Outlining of the Targets
A major potential pitfall of IMRT is the failure to select and delineate the targets accurately. This is especially relevant in head and neck cancer, where a high risk of subclinical local and nodal disease exists and where adequate irradiation of the lymph nodes at risk is crucial for local-regional control and survival. For example, in standard three-field head and neck RT, the first echelon and the retropharyngeal nodes are treated routinely when the primary tumor is targeted. In contrast, these nodes will not be adequately irradiated by IMRT if they are not specified on the planning CT.
Target Definitions in the Head and Neck
The GTVs consist of the primary tumor and lymph nodes with apparent or suspected metastasis. The primary tumor GTV is defined using radiologic and clinical assessment. Lymph node GTVs include nodes with radiologic criteria of involvement: diameter >1 cm (in the case of the jugulodigastric nodes, >1.1-1.5 cm), smaller nodes with spherical rather than ellipsoid shape, nodes containing inhomogeneities suggestive of necrotic centers, or a cluster of three or more borderline nodes.17 The clinical target volume (CTV) surrounding the primary tumor consists of tissue perceived to contain microscopic, subclinical tumor extension. In addition to the primary tumor CTV, the lymphatic CTVs consist of nodal areas that are at risk of metastatic disease but do not match the radiologic criteria of involved nodes.
Delineation of the Primary Tumor Clinical Target Volume
Factors used for assessing the extent of the CTV margins in each case include tumor site, size, stage, differentiation, and morphology (exophytic vs. ulcerative, infiltrative vs. pushing front). Rather than assign or expand the GTV uniformly, outlining of the CTV is recommended on the planning CT on slice-by-slice basis. Knowledge of the anatomic and clinical patterns of tumor extension and clinical judgment, as well as a familiarity with head and neck imaging, are necessary for accurate estimation of the CTV margins around the tumor. Specific recommendations for each tumor site have been detailed elsewhere.18
Selection and Delineation of the Lymphatic Clinical Target Volumes
Our knowledge of the pattern and risk of lymphatic drainage from different head and neck sites is based on the classical anatomic work of Rouviere,19 reviewed recently,20 the assessment of the location and prevalence of clinical neck metastasis by Lindberg et al.,21 and the large experience with elective neck dissections providing information about microscopic metastases, reported by Byers and Shah.22,23 A division of the neck to six levels has been developed by surgeons from Memorial Hospital and revised by Robbins et al.,24,25 allowing standardized and improved reporting of the nodal involvement and the surgical therapy of the neck. Adoption of this system for identification and outlining of the nodal CTVs for IMRT is highly recommended. It should be emphasized that the retropharyngeal nodes, which are not routinely dissected surgically, are not considered in the surgical neck levels classification, but are important targets in the irradiation of nasopharyngeal and other advanced head and neck cancer.26 Reviews of the risk of metastases to each neck level27 and of the neck levels at risk for each tumor site and stage18 have recently been published. Several publications are recommended for identifying the neck levels on the planning CT scans. An imagingbased nodal classification, using CT- or MRI-based criteria that correspond to the surgical anatomic landmarks, has been developed by head and neck radiologists.28 In addition, several articles have been published by radiation oncologists demonstrating how to outline the lymph node neck levels as CTVs on the planning CT scans18,27,29,30,31,32 or axial MRI.27
In the postoperative cases, the surgical specimens provide information which helps in determining the neck levels at risk. Neck dissection disrupts some anatomic landmarks used to define the borders between the levels. On the other hand, the surgical bed is apparent on the CT scan and should be encompassed entirely within the CTV.32 It is often impossible to distinguish between the primary tumor resection and the adjacent neck dissection bed, and they are encompassed within a unified CTV. Neck levels in which microscopic extracapsular lymph node extension has been found are considered high-risk volumes and are assigned a higher dose (see subsequent text). At these levels, the target may be extended to include the subcutaneous tissue.33
The Planning Tumor Volumes
Once the GTVs and CTVs are outlined on the axial CT images, a uniform expansion of these targets is performed to obtain the planning tumor volumes (PTVs) that accommodate setup uncertainties. Typically, the magnitude of the margin is 5 mm, which means that an extra 5-mm rind of normal tissue around the target receives full dose. In a region with targets and organs at risk (OARs) in close proximity, reducing this margin can potentially reduce treatment-related toxicity. Indeed, it has been estimated that each 1 mm of margin adds 1.3 Gy of dose to the parotid gland, which are particularly sensitive to low doses of radiation.34 To reduce setup uncertainty, many groups have advocated daily imaging, with a position correction if the displacement from the original plan exceeds a threshold value. At the University of Michigan, daily imaging and setup correction before each treatment fraction has reduced the setup error to 1 ± 1 mm, enabling a reduction in PTV margins to 3 mm. The importance of daily imaging and correction has been evident in studies which examined setup uncertainties: daily imaging is required if PTV margins are 3 mm, and every other day if the margins are 5 mm.35 Whether imaging is performed by CT or by orthogonal portal imaging seems to be less important than the frequency of imaging and correction.36 It should be noted that while setup uncertainties are usually measured in the upper neck, near the targets, they are frequently larger in the lower neck even if the shoulders are included in the immobilization device.
Doses are prescribed to the PTVs or to comparable “growth” areas in some commercial planning systems. When the targets are close to the skin, as may occur in postoperative case, the PTV may extend beyond the surface. In such a case, the PTV should be edited back to the surface. If the PTV extends to the skin and the skin is not at high risk, the external body contour may be defined as a noninvolved organ for the optimization system. This facilitates avoiding excessive dose to the skin.37
Similar to the expansion of the targets to yield the PTVs, there is a need to accommodate uncertainties regarding the critical normal organs, especially the spinal cord, brain stem, and the optic pathways, that may lie in regions of steep dose fall-off near the targets. This can be accomplished by expanding these organs uniformly, yielding the planning-risk volumes (PRVs). At the University of Michigan, the spinal cord is expanded by 0.3 cm to yield the spinal cord PRV. The maximal accepted doses
are 45 Gy to the spinal cord and 50 Gy to the PRV. Similarly, the optic nerves and chiasma are expanded for treatment plans of nasopharynx or paranasal sinus tumors.
are 45 Gy to the spinal cord and 50 Gy to the PRV. Similarly, the optic nerves and chiasma are expanded for treatment plans of nasopharynx or paranasal sinus tumors.
An example of the delineation of the targets and noninvolved structures in a case of oropharyngeal cancer is provided in Figure 5-1.
Dose Prescription and Specification and Normal Tissue Dose Constraints
The delivery of a single IMRT plan throughout the course of treatment provides better dose conformality compared with several consecutive plans, like the consecutive plans common in conventional radiotherapy, which consist of initial fields encompassing all targets followed with a boost to the gross tumor.38 When a single plan is prescribed, the gross tumor PTV receives both a higher total dose and a higher dose per fraction compared with the PTVs of the subclinical disease. Owing to the differences in the daily fraction doses, a correction of the total dose to yield the normalized total dose (NTD) for a 2-Gy fraction regimen is required when the fraction dose is different from standard fractionation. An extensive discussion of this issue is provided by Mohan et al.38 There are two general approaches to dose prescription. The first would be prescribing a total dose delivering standard fraction dose to the gross disease PTV, for example, 70 Gy over 35 fractions, and doses delivering low fraction doses to the subclinical disease PTVs: 63 Gy to the high-risk and 56 to 59 Gy to lesser-risk elective targets, which over 35 fractions would deliver fraction doses of 1.8 Gy and 1.6 to 1.7 Gy, yielding NTDs of 60 Gy and 50 to 54 Gy, respectively (the NTDs are calculated for late-reacting tissue, assuming an α/β of 3 Gy). When used for advanced disease, this schedule should be delivered concurrent with chemotherapy. The second strategy is to deliver a higherthan-standard fraction dose to the gross disease PTV, adjusting the total dose to yield NTD near 70 Gy, and standard fraction doses to the elective targets’ PTVs. Such a strategy was adopted by the Radiation Therapy Oncology Group (RTOG) study of IMRT for oropharyngeal cancer (RTOG study H-0022). In this study, the gross disease PTV receives a total of 66 Gy in 30 fractions, 2.2 Gy/fraction. PTVs of high-risk subclinical disease receive 60 Gy, and low-risk PTVs receive 54 Gy, at 2.0 and 1.8 Gy/fraction, respectively. This results in the gross disease PTV receiving NTD of 70 Gy over 6 weeks, similar to the total time and dose delivered by accelerated RT regimen.39 A more aggressive reported regimen delivers a total of 60 Gy in 25 fractions (2.4 Gy/fraction) to the gross disease and 50 Gy (2.0 Gy/fraction) to electively treated volumes, yielding an NTD of 66 Gy delivered over 5 weeks.40 If we take into account the relatively large target dose inhomogeneity produced by IMRT, it would be apparent that large tissue volumes within the targets receive even higher fraction doses, and higher NTDs, than those calculated for the prescribed doses. A phase I dose escalation study was conducted at the Medical College of Virginia, in which the fraction size and the total dose
to the gross disease PTVs were escalated.41,42 It was postulated that limiting the high-dose treated volume to the target alone by IMRT may reduce the risk of late complications arising from large fraction doses.40 However, critical normal tissues at risk in the head and neck, such as nerves, noninvolved mucosa, blood vessels, bone, and so on, are embedded within the targets and are at risk of late toxicity. In addition, these schemes may be associated with an excessive toxicity risk when they are combined with concurrent chemotherapy. As yet, the follow-up periods of published head and neck cancer IMRT series are not sufficient to assess the full risk of late complications arising from higher-than-standard fraction doses and total NTDs delivered to the targets. GTV dose escalation using IMRT should not, therefore, be practiced outside of careful and well-defined clinical trials.
to the gross disease PTVs were escalated.41,42 It was postulated that limiting the high-dose treated volume to the target alone by IMRT may reduce the risk of late complications arising from large fraction doses.40 However, critical normal tissues at risk in the head and neck, such as nerves, noninvolved mucosa, blood vessels, bone, and so on, are embedded within the targets and are at risk of late toxicity. In addition, these schemes may be associated with an excessive toxicity risk when they are combined with concurrent chemotherapy. As yet, the follow-up periods of published head and neck cancer IMRT series are not sufficient to assess the full risk of late complications arising from higher-than-standard fraction doses and total NTDs delivered to the targets. GTV dose escalation using IMRT should not, therefore, be practiced outside of careful and well-defined clinical trials.
![]() FIGURE 5-1. The targets outlined on planning CT in a case of stage T2, N1 carcinoma of the right tonsil, involving the lateral base of the tongue. The GTV, primary tumor CTV, and lymphatic GTV and CTVs are each expanded uniformly by 0.5 cm to yield the corresponding PTV. A: Level II nodal targets in the ipsilateral neck, and the retropharyngeal nodes bilaterally, are outlined through the base of the skull. Level II nodes in the contralateral neck are outlined up to the level in which the posterior belly of the digastric muscle crosses the jugular vein. Cephalad to this level, only the ipsilateral (LT) level II nodal CTVs and the retropharyngeal nodes are outlined. In cases where the contralateral neck contains clinical or radiologic evidence of metastasis, or in the case of nasopharyngeal cancer, level II is outlined through the base of the skull bilaterally. B: Nodal metastasis in the LT neck is outlined as a nodal GTV, expanded to yield its PTV. C and D: In the ipsilateral lower neck, nodal levels IV and V are included in the CTV. Only level IV is outlined in the contralateral lower neck (the contralateral neck did not have clinical evidence of metastasis). E: A sagittal reconstruction of the CT may help in the assessment of the extent of disease. On axial images, it is difficult to distinguish the soft palate from the base of tongue that contains the targets. The sagittal view facilitates the exclusion of the soft palate (P) from the targets. CT, computed tomography; GTV, gross tumor volume; CTV, clinical target volume; PTV, planning tumor volumes. (See color insert.) |
Dose and dose-volume specifications are made to impose constraints on the DVHs of the targets, noninvolved tissue of interest, and nonspecified tissue outside the targets. RTOG protocol H-0022 specified the prescription dose as the dose that encompasses at least 95% of the PTV. No more than 20% of the PTV can receive >110 % and no more than 1 % of the PTV can receive <93 % of the prescribed dose. To limit hot spots outside the targets, the protocol specifies that no more than 1% of the tissue outside the PTVs can receive >110% of the prescribed dose. This is done by adding a dose constraint for all nonspecified tissue (all tissue outside the targets and the specified organs) to prevent volumes of high dose outside the targets.
Dose constraints regarding critical organs are usually stated in terms of the maximal dose. Commonly applied constraints in the head and neck are maximal doses of 45 Gy to the spinal cord, 54 Gy to the brain stem, 70 Gy to the mandible, and 50 to 55 Gy to the optic pathway. These constraints were derived from standard irradiation, where the organ at risk receives irradiation at a
standard daily fraction dose for part of the therapy course and is then fully shielded. In contrast, IMRT typically delivers lower daily fraction doses to these organs throughout therapy. In addition, the maximal dose derived from the DVH represents only a small organ volume, whereas standard RT delivers any specified dose homogeneously to relatively large volumes. In most instances, therefore, the same dose constraints are more conservative when applied to IMRT compared with standard RT. On the other hand, steep fall-off of doses near the critical organs may increase the risk of inadvertent overdosage to these organs due to motion and setup uncertainties. This issue can be addressed by a uniform expansion of the critical organs to yield the PRVs, as discussed in the preceding text. Organs with parallel functional architecture require specification of the mean dose or partial organ volume dose, rather than the maximal dose. Examples are the specification in RTOG protocol H-0022 of the maximal mean dose to the parotid salivary glands at 26 Gy, or limiting the dose to at least 50% of the gland volume to <30 Gy, and constraining the dose to two thirds of the larynx at <50 Gy.
standard daily fraction dose for part of the therapy course and is then fully shielded. In contrast, IMRT typically delivers lower daily fraction doses to these organs throughout therapy. In addition, the maximal dose derived from the DVH represents only a small organ volume, whereas standard RT delivers any specified dose homogeneously to relatively large volumes. In most instances, therefore, the same dose constraints are more conservative when applied to IMRT compared with standard RT. On the other hand, steep fall-off of doses near the critical organs may increase the risk of inadvertent overdosage to these organs due to motion and setup uncertainties. This issue can be addressed by a uniform expansion of the critical organs to yield the PRVs, as discussed in the preceding text. Organs with parallel functional architecture require specification of the mean dose or partial organ volume dose, rather than the maximal dose. Examples are the specification in RTOG protocol H-0022 of the maximal mean dose to the parotid salivary glands at 26 Gy, or limiting the dose to at least 50% of the gland volume to <30 Gy, and constraining the dose to two thirds of the larynx at <50 Gy.
Recommended dose constraints to specific organs are beyond the scope of this chapter. A recent effort by multiple clinicians and physicists, Quantitative Analysis of Normal Tissue Effects in the Clinic (QUANTEC), resulted in the publications of papers detailing normal tissue toxicity probabilities for critical organs based on actual data accumulated in the past decade.43 These publications aimed specifically at guiding IMRT dose prescriptions and constraints for each critical organ.
The Optimization Process
The PTVs and uninvolved organs lie in close proximity or overlap with each other. It is necessary to assign weighting factors (or penalty/importance factors) to each target and organ, which determine the relative importance of fulfilling their dose specifications or constraints. These weighting factors are derived following an iterative, trial-and-error process that requires refinement for each patient in order to produce an optimal plan. These factors differ among the various optimization systems. Examples of penalty factors for head and neck cancer plans were provided by several authors.44,45 It was noted that because normal structure doses are penalized during optimization only if they exceed the limits set by the user, the constraints need to be more stringent than the clinical criteria.45 At the University of Michigan, the optimization system uses a cost function that strives to minimize the dose to some noninvolved structures, in addition to setting a maximal dose constraint. This facilitates a reduction of the doses to these organs as much as possible, rather than settling at just below the maximal dose allowed.46 Also, using a combination of linear and high-power objective functions (in addition to the quadratic objective function used in most commercial systems), strict head and neck target dose homogeneity can be achieved.46
In addition to the physical dose and dose-volume optimization criteria, several investigators examined the utility of biologic/clinical criteria as a basis for optimization in the head and neck. These investigations include optimization using the probability of uncomplicated tumor control,47 tumor control probability (TCP) and normal tissue complication probability (NTCP),48 and the equivalent uniform dose (EUD) concept, which is defined as the biologically equivalent dose that, if given uniformly, will lead to the same cell kill in the tumor volume as the actual nonuniform dose distribution.49 The latter investigators found that optimization using EUD as the cost function is superior to optimization using dose or dose-volume. Biologic cost functions are expected to be superior to dose-based functions when the parameters of the biologic models, derived from clinical dose-response and dose-complication data, are known with greater confidence. An example is the optimization of advanced paranasal sinus cancer plans, where optic pathway NTCPs derived from patient complication data were used for the critical organ cost function.50
The Number and Directions of The Beams
While tomotherapy uses arcs around the patient, IMRT using MLCs requires decisions regarding the number and orientation of the beams. It has been suggested early on that if the number of segments (or beamlets) is large enough, the direction of the beams is not important, and coplanar beams arranged at equidistance around the patient’s head and neck would achieve satisfactory results. Most investigations of IMRT of head and neck with MLCs use this approach. The beam number should be odd, to prevent opposed beams which would increase hot spots near their entrance to the neck. Nine beams arranged at equidistance (40° apart) were found to be optimal: they provided better dose distributions than 5 or 7 beams, whereas 15 beams did not seem to improve the plans.41 An optimization of the beam angles was found to be unnecessary by some authors,41 whereas others reported an improvement in head and neck plans when optimized, noncoplanar beam angles were used.51 This issue continues to be a subject of research. Current recommended field arrangement for IMRT of head and neck cancer with MLCs is nine equidistant, coplanar fields (Fig. 5-2), for patients with advanced tumors and complex anatomy like nasopharyngeal cancer. On the other hand, patients with prior tumors, or less complex anatomy, seven or even five coplanar nonopposed fields may achieve the planning objectives.
In patients without nodal disease in the lower neck, in whom the primary tumor resides in the nasopharynx, oropharynx, or oral cavity, treating the upper neck and primary tumor with IMRT while abutting the IMRT fields to an anterior-posterior low field containing a laryngeal block (split-field IMRT) reduces monitor units. It also reduces contouring and treatment time and can achieve better sparing of the larynx compared with whole-field IMRT.52 However, if glottic larynx sparing is denoted by a higher weight (importance) in the optimization than full coverage of the
targets in the low neck, laryngeal avoidance can be as good.53 The benefits of whole-field IMRT are mostly in cases with high-risk targets in the lower neck, where this method ensures adequate target coverage, while patients whose low-neck targets are at low risk are best treated with split-field IMRT.
targets in the low neck, laryngeal avoidance can be as good.53 The benefits of whole-field IMRT are mostly in cases with high-risk targets in the lower neck, where this method ensures adequate target coverage, while patients whose low-neck targets are at low risk are best treated with split-field IMRT.
![]() FIGURE 5-2. Beam arrangement for IMRT using an MLC. Nine coplanar, equidistant beams are used, each containing 1 × 1 cm or 0.5 × 0.5 cm pencil “beamlets.” IMRT, intensity-modulated radiotherapy. (See color insert.) |
Clinical Results of IMRT Series: General
Most series reporting clinical results of tumor control rates following IMRT are still quite limited. They are either very heterogeneous regarding tumor sites and stages26,31,33,54,55 have relatively small patient numbers or suffer from relatively short follow-up. Potential patient selection factors are probably the most important issues that should be considered in assessing the outcome in retrospective series of IMRT. IMRT is far more complex and time consuming compared with conventional radiotherapy. It also requires extensive skill and time commitment on the part of the radiation oncologist. It is likely that different selection factors play a role in each institution: patients who cannot tolerate lengthy treatment, those judged to be too sick to benefit from complex therapy, those requiring urgent start of therapy, and so on, may be selected to receive more simple, conventional treatment. These factors make any attempt to compare the results of different IMRT series, or even series of IMRT to series of conventional RT, futile. These issues have been demonstrated in a recent analysis from the University of Wisconsin, which assessed potential selection factors for patients treated with IMRT or conventional RT during the same time period.56 They found that patients with T3-4 and N3 disease were less likely to be treated with IMRT. IMRT was found to be a significant prognostic factor improving tumor control rates; however, when the T stage was included in the model, the use of IMRT lost independent significance. Notably, although treatment results using IMRT were better than the results in the pre-IMRT era, there was also an improvement of tumor control rates in patients treated with conventional RT in recent years compared to those treated with conventional RT in the early 1990s.56 Therefore, tumor control improvements in recent years may be related to factors like improvements in tumor imaging and chemotherapy, rather than solely to the introduction of IMRT. Because the use of IMRT becomes more common and the large majority, or all patients, at each institution are treated with IMRT, the potential bias due to patient selection factors in clinical series of IMRT is diminishing.
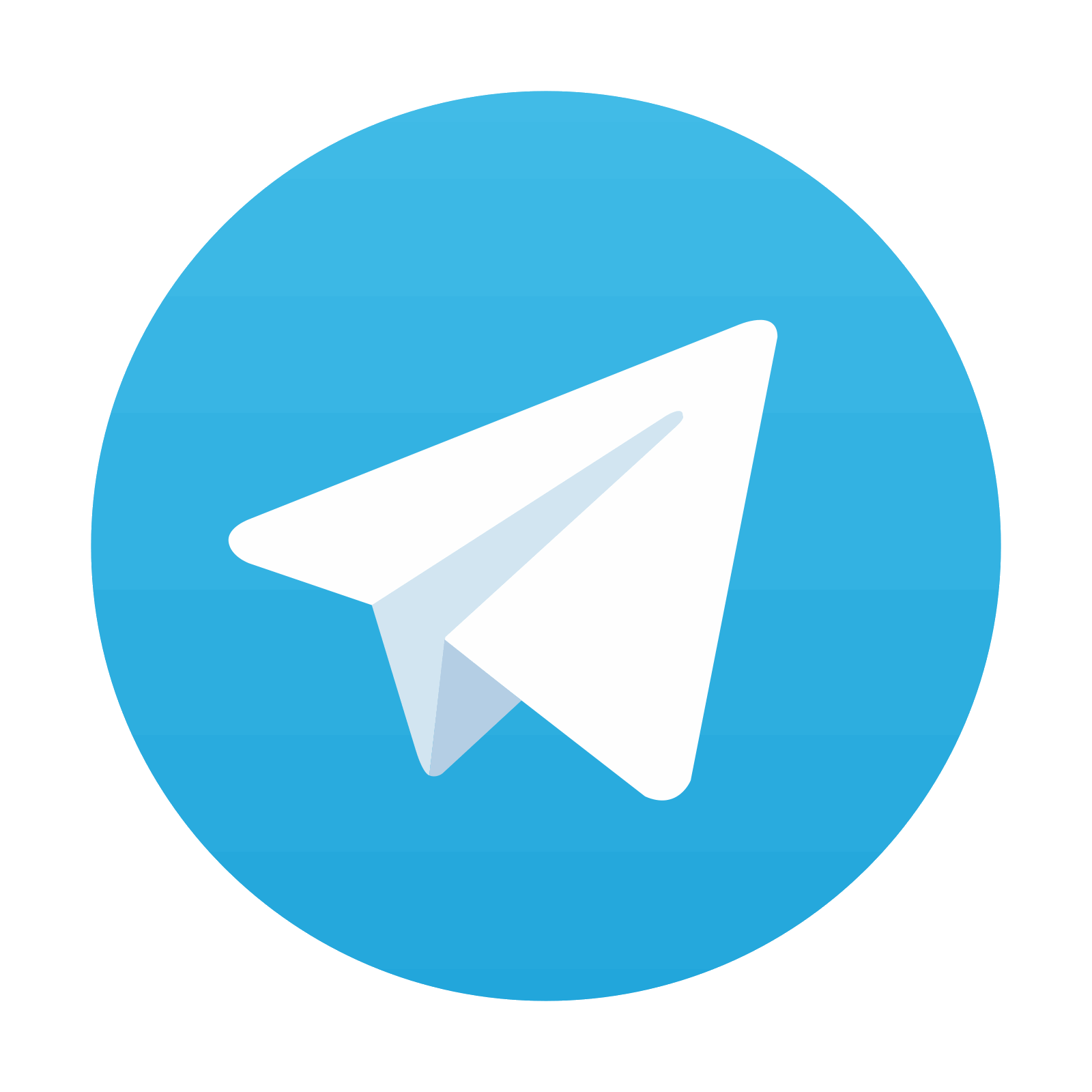
Stay updated, free articles. Join our Telegram channel
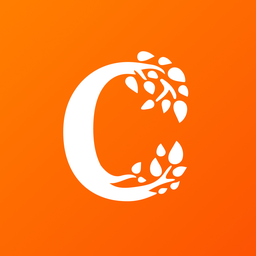
Full access? Get Clinical Tree
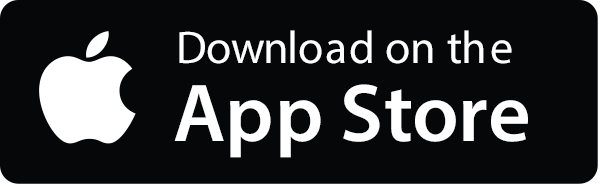
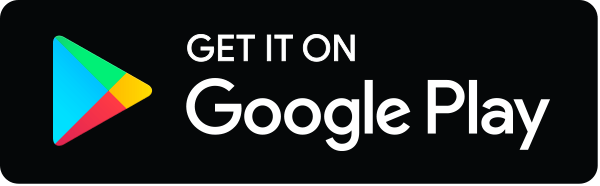