Introduction
The brain controls the eyes to ensure optimal visual acuity as we navigate our environment throughout our daily lives. First, we make voluntary eye movements to explore our visual world and shift our focus attention from one object to another. Second, we can also voluntarily track an object as it moves through our environment. And third, as we move through space, our brains generate reflexive eye movements that reduce the motion of the visual surround relative to both retinas to ensure gaze stability. How the brain controls eye movements during all of these conditions is well understood, both in terms of the underlying neurophysiological mechanisms and anatomical organization.
Eye movements can be categorized into five classes ( Table 8.1 ). Of these, three classes are voluntary eye movements made to redirect our gaze relative to the world. These voluntary eye movements include saccades, smooth pursuit, and smooth vergence. Specifically, saccades , which derive their name from the French word for “jerk,” are rapid transient eye movements. Saccades are made to voluntary redirect gaze between stationary objects in the visual environment. By precisely positioning each eye, saccades effectively target the fovea so that an object can be viewed with the greatest acuity . Smooth pursuit eye movements are also foveating voluntary eye movements. However, in contrast to saccades, smooth pursuit eye movements are relatively slow and are made to track moving objects rather than shift gaze from one stationary object to another. Finally, animals with binocular vision such as humans make smooth vergence eye movements to track a target moving in depth and/or maintain fused binocular vision on a target.
Eye movement classification | Function |
---|---|
Voluntary | |
Saccades (conjugate/ disjunctive) | Fast gaze redirection between stationary far targets/stationary targets in depth |
Smooth Pursuit | Slow eye movements used to track moving targets |
Smooth Vergence | Slow eye movements used to track an object moving in depth/maintain fused binocular vision |
Involuntary | |
Vestibulo-ocular reflex | Uses vestibular information to hold images stationary on the retina as head moves |
Optokinetic reflex | Uses visual information to stabilize gaze for low frequency head movements |
The two remaining classes, namely the vestibulo-ocular reflex (VOR) and optokinetic reflex (OKR), are reflexive rather than voluntary eye movements. VOR and OKR eye movements are made to stabilize (rather than voluntarily redirect) gaze direction relative to the world. Specifically, the VOR functions to keep the visual world stationary on the retina during everyday activities such as walking and running by moving the eye in the opposite direction to the on-going head motion. Head motion is detected by the vestibular sensory organs of the inner ear, which in turn transmit commands to the brain that produce compensatory eye motion so that the visual world remains stationary on the retina. Correspondingly, the OKR also functions to stabilize gaze during everyday activities such as walking and running. However, in contrast to the VOR, OKR eye movements are driven by activation of the visual rather than vestibular system. The visual input from a large moving scene subtending much or all the visual field produces compensatory OKR eye movements that function to keep the visual scene stationary on the retina. The VOR is most effective for head motion at frequencies above 0.1 Hz, whereas the OKR reflex is most effective at lower frequencies, particularly below 0.1 Hz. Thus, in everyday life the VOR and OKR are complementary and work synergistically to stabilize gaze in response to head movements.
Traditionally, neurophysiological and behavioral experiments have studied and characterized each of the five classes of eye movements in isolation. As a result, as detailed in sections “Final common pathway” and “Premotor control of gaze redirection,” we have an excellent understanding of the oculomotor pathways that generate each class of eye movement ( Table 8.1 ). However, this experimental approach has also led to the prevailing (and incorrect) view that each of eye movement class is generated by separate and distinct neural pathways. Indeed, as discussed in section “Interactions between eye movement subsystems” the brain typically simultaneously employs two or more oculomotor “subsystems” to control gaze, and, most notably, vergence is generated by the same oculomotor pathways that generate saccades and smooth pursuit.
Final common pathway
To voluntarily move the eyes, a hierarchy of neural control exists within each of the functional categories of eye movements that plans, coordinates, and executes motor activity ( Box 8.1 ). First, high-level structures, including regions of the cortex, basal ganglia, and cerebellum, underlie cognitive aspects of eye movements including visual attention and decision-making to plan where to next focus gaze ( Box 8.1 ). Integration of such higher-level input by structures such as the superior colliculus is then required to trigger the planned eye movement. The superior colliculus activates premotor pathways that activate the motoneurons that control the extraocular muscles, which in turn move each eye about its center of rotation. These motor neurons are located in the three cranial nuclei that comprise the “final common pathway” for all classes of eye movements. Premotor pathways in the brainstem that mediate saccades, smooth pursuit, vergence, VOR and OKR eye movements all send the required motor commands to these motoneurons to generate eye movement. The next section, “The extraocular eye muscles and their motoneuron innervations,” describes the final common pathway for all eye movements.
Subcortical oculomotor disorders are classified by lesion sites in the hierarchy of motor control:
- •
Supranuclear (motor planning stage)
- •
Internuclear (connections between nuclear and premotor sites)
- •
Premotor (coordinates combined actions of several muscles)
- •
Nuclear (cranial motor nuclei making up the final common pathway)
- •
Peripheral (cranial nerves and muscles)
The extraocular eye muscles and their motoneuron innervations
To ensure a clear unified view of the world during our everyday activities, the brain must precisely coordinate the movement of our eyes so that both foveae are aligned on the same point in space. To do this the brain activates the six extraocular muscles that control the eye’s movement and position. The six extraocular muscles are arranged in three antagonistic pairs ( Fig. 8.1A ) that together generate the net force required to rotate the eye to a new position. First, the relative activation of the paired lateral and medial recti muscles controls horizontal eye movement (i.e., the temporal-nasal rotation of each eye, respectively). Second, the relative activation of the paired superior and inferior recti muscles principally controls vertical movement (i.e., the up-down rotation [elevation] of each eye, respectively). Third, the relative activation of the paired superior and inferior oblique muscles controls the torsional rotation of each eye, as well as its elevation. Together, these three pairs of extraocular muscles allow the eye to rotate with three degrees of freedom ( Fig. 8.1B ). The VOR and OKR operate fully in three dimensions, while orbital mechanics and central mechanisms fundamentally restrict saccadic and smooth pursuit eye movements to two dimensions. This constraint on three-dimensional voluntary eye movements is referred to as Listing’s law (see Chapter 9 ).

To activate each of these six extraocular muscles, the brain sends commands to the motoneurons located within the three oculomotor cranial nerve nuclei ( Fig. 8.2A , shaded red structures). These cranial nerve nuclei include the oculomotor (III), trochlear (IV), and abducens (VI) nuclei of the mesencephalon and pons. Notably, the oculomotor (III) nucleus is located in the midbrain and comprises four distinct anatomical regions in which motoneurons specifically control either the medial, superior or inferior recti, or inferior oblique. The trochlear (IV) nucleus is also located in the midbrain and its motoneurons specifically control the superior oblique muscle. And finally, the abducens (VI) nucleus, which is located in the pons, controls the lateral rectus muscle. A shared feature across all three cranial nerve nuclei is that, in all cases, any given motoneuron only projects to a single extraocular muscle.

Additionally, some neurons within the cranial nerve nuclei controlling horizontal eye movements are actually not motoneurons, but instead project to the motoneurons controlling the antagonistic muscle of the other eye. These neurons, which are called internuclear neurons, encode similar eye movement-related information, as do the motoneurons. Thus, internuclear neurons are thought to facilitate the neural control of conjugate component of binocular eye movements, namely the component of the eye movement that is common to both eyes. For example, internuclear neurons within the abducens and oculomotor nucleus project via the medial longitudinal fasciculus (MLF) to the contralateral oculomotor and abducens nucleus, respectively. , Thus, the motoneurons of the left abducens nucleus project directly to the left lateral rectus, while the internuclear neurons of the left abducens project contralaterally to motoneurons in the right oculomotor (III) nucleus ( Fig. 8.2B ). The synchronous activations of both cell types thus serve to generate complementary temporally directed movement of the left eye and nasally directed movement of the right eye, such that both eyes effectively move in the same (i.e., leftward) direction. Correspondingly, the synchronous activations of motoneurons and internuclear neurons from oculomotor nucleus would function to generate complementary nasally directed movement of the left eye and temporally directed movement of the right eye, such that both eyes effectively move in the same (i.e., rightward) direction.
Finally, it is noteworthy that there are two unique features of extraocular muscle. First, each of the six extraocular muscles is comprised of two distinct layers, a global layer and an orbital layer. These two layers contain fibers with different response properties (e.g., fatigability, fusion frequency, and contraction times; see Chapter 7 ) and in comparison to the muscles of the skeletal system, extraocular muscles in both the global and orbital layers have relatively fast contraction times. , Twitch fibers appear to receive stronger innervation from premotor pathways involved in the generation of fast eye movements (e.g., the saccadic burst generator), whereas nontwitch fiber innervation from premotor sources is involved in executing slow eye movements (e.g., pursuit, the VOR, OKN). , Second, in contrast to skeletal muscle, human extraocular muscles have muscle spindles but not Golgi tendon organs. In addition, extraocular muscles have a unique class of receptors—palisade endings—which are believed to serve as proprioceptors. Importantly, although human extraocular muscles process proprioceptor receptors, there is no evidence for a stretch reflex as is observed in skeletal muscle. The role of these receptors in extraocular muscles remains to be fully understood; however, there is reason to believe that they signal proprioceptive information from the extraocular muscles to the brain to ensure accurate visuomotor behavior. ,
The biomechanics of eye movements and motor neuron activity
Mechanics of the oculomotor plant
To move the eye to a new position, the extraocular muscles must generate the active force necessary to overcome the passive restraining forces of the eyeball, extraocular muscles, and supporting tissues of the orbit. In his pioneering work, David Robinson established that these passive forces are predominately governed by viscoelastic properties. Specifically, the eye’s natural tendency to “spring” back to its center position when moved right/left or up/down defines its passive elasticity, whereas the finding that more force is required to move the eye at faster and faster velocities defines the eye’s passive viscosity. In this same study, Robinson further made the surprising discovery that the eyeball has negligible inertia. Combined, the viscoelastic properties of the eye’s passive restraining forces are central to our understanding of how motoneurons control eye movements. Notably, because the eyeball and its orbital tissues are dominated by viscoelastic properties and have negligible inertia, the active force required to move the eye can be well approximated by the simple first-order differential equation of the form:
where F is the force, k is the coefficient of elasticity, L is the length of the muscle, r is the coefficient of viscosity, and dL/dt is the rate of muscle length change (i.e., velocity). Given the anatomical complexity of the eyeball, extraocular muscles, and supporting tissues of the orbit, it is remarkable how well its mechanics are represented by such a simple equation. For example, Eq. 8.1 predicts that, when a constant external force is applied to the eye and then removed, the eye will passively drift back to the center position following a simple exponential characterized by the time constant r/k. Indeed, experiments confirming this prediction have established that the time constant of the return to center is around 250 ms ( Fig. 8.3 ).

The relationship between motoneuron activity and eye movement
The simple relationship between force and eye movement represented by Eq. 8.1 has proven to be advantageous for understanding the neural control of eye movements. This is because motoneuron discharge is well related to active muscle force. Neural recordings made from individual extraocular motoneurons in alert-behaving monkeys demonstrated that the response of a given motoneuron, like force, is well approximated by Eq. 8.1 . This point is demonstrated in Fig. 8.4 , which illustrates the activity of a typical inferior rectus motoneuron in different conditions. First, when the monkey held its eye stationary, approximately centered in the orbit (i.e., position = 0 degrees), the motoneuron discharged at a constant firing rate—namely its resting discharge ( Fig. 8.4A : top, red arrow). Second, when the monkey made downward and upward eye movements to fixate target ( Fig. 8.4A , top), the example motoneuron’s firing rate increased and decreased, respectively, consistent with the pulling direction of the inferior rectus. The example motoneuron is typical in that its sustained change in firing rate was proportional to eye position—in this case, vertical eye position ( Fig. 8.4A : bottom, red line). This same relationship is plotted for other example neurons in blue in Fig. 8.4A . Finally, the example extraocular motoneuron firing rate response was also related to eye velocity. This can be appreciated in Fig. 8.4B , in which the example neuron’s activity is shown during vertical smooth pursuit eye movements. Comparison of the neurons’ responses when the eye moves past the same orbital position reveals that firing rate is linearly related to the current velocity ( Fig. 8.4B , red arrows).

Combining the findings of the experiments in Fig. 8.4 results in a first-order differential equation that well describes the relationship between motoneuron responses and eye movement across each of these three conditions:
where FR(t) is the neuron’s instantaneous firing rate, b , k , and r are constants that represent the neuron’s resting rate when the eyes are pointing straight ahead (mean firing rate), and the neuron’s eye position (slope of Fig. 8.4A , bottom) and eye velocity sensitivities (slope of Fig. 8.4B , bottom), respectively. Note b can take on negative values for motoneurons that are only recruited at eccentric eye positions in their preferred direction. t d refers to the dynamic lead time, and E (t) and Ė(t) refer to the instantaneous eye position and velocity, respectively.
Importantly, Eq. 8.2 is of the same form as Eq. 8.1 , which described the biomechanics of the eye. Furthermore, the ratio characterizing average motoneuron discharges (r/k, Eq. 8.2 ) well approximates the 250-ms time constant of the extraocular plant (r/k, Eq. 8.1 ). In short, motoneuron discharge dynamics are proportional to the active force generated to move the eye, and thus effectively matched to the eye’s biomechanics. Moreover, Eq. 8.2 can be used to describe eye movements not only during steady fixation, ocular fixation, and smooth pursuit, but also during all other eye movements, including the VOR and smooth vergence. However, the parameters in Eq. 8.2 that correspond to motoneuron eye velocity and position sensitivities (i.e., r and k) decrease as a function of the eye’s velocity. , Specifically, a given motoneuron’s eye velocity and position sensitivities are greater for slower movements (e.g., pursuit) compared with faster movements (e.g., saccades). , This is likely due to nonlinearities in biomechanics of the extraocular plant that are generated by the velocity-dependent viscosity of the extraocular muscles. As a result, faster eye movements such as saccades are ultimately met with less viscous resistance such that a smaller change in force (i.e., corresponding to smaller coefficients) is required to move the eye.
A pulse-step command is required to control saccadic eye movements
As explained previously, a simple first-order differential equation ( Eq. 8.1 ) well describes the biomechanics of the eye. These dynamics have important implications regarding the motor commands that must be generated by the motoneurons to generate eye movements. For example, one might intuitively guess that a step change in motoneuron firing rate would be sufficient to generate a saccadic eye movement. However, this is not the case. Even large redirecting eye movement (i.e., amplitude >40 degrees) can be completed in less than 100 ms. In contrast, the dominant time constant of the biomechanics of the eye ( Eq. 8.1 ) is around 250 ms. What this means is that a step change in motoneuron firing rate would produce an eye movement that would still be around 37% short of its final position after around 250 ms.
Consequently, a step change in motoneuron firing rate is not sufficient to generate a saccadic eye movement. Instead, to generate saccades that move the eye to its final desired position in this shorter time frame, motoneurons must generate a burst (or “pulse”) of action potentials. This burst of activity is required to offset for the eye’s passive viscosity, represented by the eye velocity-dependent term (rE’) in Eq. 8.2 . Fig. 8.5A illustrates a schematic of the saccadic pulse-step command signal sent by motoneurons to extraocular muscles. Single unit recording studies in primates have demonstrated that the saccade-related bursts of motoneurons reach rates as high as 500 spikes/s. Once the saccade has been completed and the eye is oriented to focus on a new target of interest, motoneurons indeed continue to generate a sustained constant firing rate (or “step”) to keep fixed in any eccentric location. This step of activity is required to offset for the eye’s passive elasticity, represented by the eye position dependent term (kE) in Equation 2. Notably, this “pulse-step” pattern of motoneuron activity is evident in Fig. 8.4A . In this typical example, an inferior rectus motoneuron first produces a burst of spikes (the “pulse”) to generate downward saccades. After this saccade-related burst, the same motoneuron then produces a sustained constant firing rate (or “step”) which holds the eye stationary at this new location, to prevent it from drifting back to its centered location in the orbit. Correspondingly, as a result of this motoneuron pulse-step command signal, extraocular muscle tension first rises to a peak and then rapidly decays to a steady state level ( Fig. 8.5B ).

Premotor control of gaze redirection
Having examined the properties of the motor neurons that control eye movements, the premotor circuits that command each class of eye movement when performed in isolation are now considered. This section will focus on the premotor pathways in the brainstem that mediate voluntary eye movements that are used to redirect the direction of gaze, namely saccades and smooth and vergence eye movements.
Neural control of saccadic eye movements
Saccades are rapid eye movements that are made to redirect the fovea to fixate a target of interest ( Fig. 8.6 ). When exploring the visual world, humans routinely make two to three saccadic eye movements each second. Larger amplitude saccades are characterized by higher velocities, with the largest saccades reaching velocities up to 700 degrees/s for 40-degree saccades in humans. The consistent relationship between the saccadic duration, peak velocity, and amplitude is referred to as the “main sequence” ( Box 8.2 ). For example, the relationship between amplitude and peak velocity is well described by a power law function. , Approximately 200 milliseconds are required to initiate a saccade in response to the appearance of an unexpected visual target.

Deviations from the main sequence (i.e., the relationships between duration, peak velocity, and amplitude of human saccades) can be used in patients to identify abnormalities in oculomotor pathways.
Pathway overview
Higher-level structures, including regions of the cortex and cerebellum, play an essential role in visual attention and the decision-making process regarding where to next direct gaze. Integration of such higher-level input by structures such as the superior colliculus is then required to trigger the next saccadic eye movement. The superior colliculus, a bilateral structure located on the roof of the midbrain, plays an especially important role in visuo-oculomotor integration required for controlling saccades. This midbrain region effectively serves as an information “hub” that receives inputs from multiple cortical areas, including the frontal eye fields, posterior parietal cortex, and basal ganglia ( Fig. 8.7 ). Cells in the deeper superior colliculus layers are arranged in a functional “motor map,” with a series of iso-amplitude lines which run medial-laterally and a series of iso-direction lines that run rostral-caudally ( Fig. 8.8 ).


Output neurons in these deeper layers of the superior colliculus send direct contralateral projections to three structures within the brainstem, which correspond to key nodes in the brainstem saccadic circuit, namely the paramedian pontine reticular formation (PPRF), rostral interstitial nucleus of medial longitudinal fasciculus (riMLF), and raphe interpositus nucleus (RIP). The caudal superior colliculus preferentially targets the PPRF and riMLF, regions containing premotor saccadic burst neurons that project to the extraocular motoneurons to generate the horizontal and vertical/torsional components of saccadic eye movements, respectively. In contrast, the output neurons in the deeper layers of the rostral superior colliculus send direct projections to the RIP. As discussed further in the chapter, the nucleus raphe pontis contains omnipause neurons that pause for saccades made in all directions. Accordingly, as shown in Fig. 8.9 , the superior colliculus plays a role in both the generation of saccades and fixation via its caudal versus rostral connectivity to brainstem premotor circuits; neurons in the caudal superior colliculus trigger saccades, whereas those in the rostral superior colliculus have an active role in maintaining fixation.

The superior colliculus’ functional “motor map” was initially defined by microsimulation: Stimulating increasingly caudal sites produces larger and larger contralateral saccades, whereas stimulating sites that are increasing medial versus lateral to the midline produce saccades that are increasing downward versus upward (reviewed in ). Individual superior colliculus neurons display saccade-related burst activity with direction/amplitude tuning that correspond to their location on the motor map. Further, this saccadic burst activity predominately encodes motor, not sensory, information—neurons respond to saccadic eye movement of a particular amplitude rather the appearance of a target at a particular location on the retinal map. A large population of coarsely tuned superior colliculus neurons comprise a “hill” of activity that drives a given saccade. If one only had access to the information encoded by a single superior colliculus neuron, there would be ambiguity in coding of both saccade amplitude and direction. Instead, the direction and amplitude of a saccadic movement is read out from this population of neurons. Finally, in addition to their descending projections to brainstem regions controlling eye movements, the output neurons of the superior colliculus have ascending projections that target forebrain structures including the basal ganglia and amygdala. Through these pathways, the superior colliculus also contributes to visual perception and cognition, for instance, playing an essential role in attention and decision-making, as well as motor control (reviewed in Zenon and Krauzlis, 2014; Basso et al., 2021 , ).
The superior colliculus sends descending projections to brainstem structures that produce the “pulse-step” motoneuron command required to generate saccadic eye movements (see section “A pulse-step command is required to control saccadic eye movements”). Specifically, the “pulse-step” command is the consequence of the integration of two distinct premotor inputs at the level of the extraocular motoneurons ( Fig. 8.9 ). For the horizontal component of saccades, premotor burst neurons in the PPRF provide the “pulse” command, while premotor neurons in the nucleus prepositus (nPH) provide the “step” command. A complementary and separate parallel circuit controls the vertical/torsional component of saccades; the pulse is provided by vertical burst neurons in the riMLF and the step is provided by premotor neurons in the interstitial nucleus of Cajal. This premotor saccadic circuitry is considered in more detail further for both the horizontal and vertical/torsional components of saccades.
The pulse of the “pulse-step” command for horizontal saccades
The premotor circuit generating the “pulse” command for the horizontal component of saccades is very well understood. To drive horizontal saccades, two classes of saccadic burst neurons work together: excitatory and inhibitory burst neurons. Excitatory burst neurons are located in the rostral PPRF and excite the agonist motoneurons (i.e., ipsilateral abducens), while inhibitory burst neurons are located in the caudal PPRF and inhibit the antagonist motoneurons (i.e., contralateral abducens) (reviewed in , ). Both excitatory and inhibitory saccadic burst neurons fire a burst of action potentials that precedes saccade onset by approximately 10–20 ms, but are otherwise silent. For example, these neurons are silent during steady gaze, smooth pursuit, and smooth vergence, and during the slow phases of vestibular nystagmus.
The burst activity of both classes of saccadic burst neurons well correlates with measures of the saccadic movement ( Fig. 8.10 A,B ). Specifically, the duration of the burst and saccade are well correlated, as are the number of spikes within a burst and saccade amplitude. , , Furthermore, the pattern of action potentials in the burst dynamically encodes saccadic eye velocity as a function of time. , , , Overall, the same first-order model for extraocular motoneurons discussed previously in the chapter ( Eq. 8.2 ) can also be used to describe the time-varying saccadic burst neuron activity, by setting the position sensitivity to zero (i.e., k = 0). These response dynamics are consistent with the role of saccadic burst neurons in producing the “pulse” of the pulse-step command. Owing to its role in commanding horizontal saccades, chemical inactivation of the PPRF results in a substantial reduction in peak velocity and proportional increase in duration for ipsilateral saccades.

The step of the “pulse-step” command for horizontal saccades
To hold the eye steady at its new horizontal location following a saccade, the nucleus prepositus sends a “step” command directly to the extraocular motoneurons (reviewed in ). The mechanism by which this premotor control of “step” command is generated for horizontal saccades is also well understood. The nucleus prepositus receives direct input from the saccadic burst neurons in the PPRF. The nucleus prepositus in turn generates the “step” command by integrating (in the mathematical sense) its burst neuron input. Neural integration is performed within the nucleus prepositus itself via recurrent connectivity between individual neurons that results in correlated firing activity between single neurons that varies as a function of distance. ,
As a result of this integration, neurons in the nucleus prepositus predominately send a horizontal eye position signal to the extraocular motoneurons. Thus, the same first-order model for motoneurons discussed previously in the chapter ( Eq. 8.2 ) can also be used to describe nucleus prepositus neuron activity, where the position sensitivity (i.e., k) dominates, consistent with the role of these neurons in producing the “step” of the pulse-step command. In short, because the burst neuron “pulse” command encodes eye velocity, integration of this input within the nucleus prepositus effectively produces an eye position—or equivalently the required “step”—command. Owing to its role in controlling horizontal saccades, lesions of the nucleus prepositus result in the inability to hold the eyes at a new horizontal position after a saccade.
The vertical/torsional “pulse-step” command for saccades
As noted previously, a parallel circuit, complementary to that described for the horizontal component of saccades, controls the vertical/torsional component of saccades. First the “pulse” command is provided by premotor neurons in the mesencephalic reticular formation (MRF). Specifically, individual neurons in the riMLF burst for upward or downward saccades, but are otherwise silent. , These burst neurons send this pulse of the “pulse-step” saccade command directly to the motoneurons in the oculomotor and trochlear nuclei that control vertical/torsional eye movements. Additionally, these burst neurons also project to the interstitial nucleus of Cajal, which integrates the pulse signal to produce the required “step” command. , In turn, interstitial nucleus of Cajal neurons send the step command directly to the motoneurons controlling the vertical/torsional eye motion. Accordingly, lesions of the riMLF and interstitial nucleus of Cajal lead to the impaired generation of vertical and torsional saccades. , , These results show that burst neurons in the riMLF play a decisive role in generating rapid eye movements with a vertical and torsional component.
Together the riMLF and interstitial nucleus of Cajal generate the pulse and step commands required to control the vertical/torsional component of saccades. As a result, this premotor circuit and the parallel horizontal circuit described previously provide the required “pulse-step” input for saccades in all directions. It is noteworthy that the neural integration of the PPRF and riMLF pulse command by the nucleus prepositus and interstitial nucleus of Cajal is imperfect, such that the eyes will very gradually drift back to center position with a time constant of approximately 25 s following a saccade. However, this is typically not a major issue for visual stability, given that several saccades are made within each second during normal viewing.
The role of omnipause neurons
Omnipause neurons function to actively inhibit the generation of saccadic eye movements during fixation. They are located near the midline in the RIP, close to the rostral pole of the abducens nucleus. While the output neurons within the caudal superior colliculus activate saccadic burst neurons in the PPRF and central mesencephalic nucleus to control saccades, the output neurons of the rostral zone of the superior colliculus are tonically active during fixation and preferentially activate omnipause neurons. Omnipause neurons, in turn, send direct inhibitory projections to saccadic burst neurons in the PPRF and in the MRF. Consequently, omnipause neurons actively suppress the horizontal and vertical/torsional premotor saccade pathways during fixation. Correspondingly, omnipause neurons receive reciprocal inhibition from saccade burst neurons in the PPRF during saccades. , ,
The duration of an omnipause neuron’s pause is well correlated to saccade duration. , Further, the hyperpolarizations that occur in association with saccade-related pauses similarly encode eye movement velocity for saccades in all directions ( Fig. 8.10C , ). The role of omnipause neurons in active fixation and the inhibition of saccadic eye movements has been causally demonstrated in experiments showing that microsimulation of the omnipause region of the RIP causes complete cessation of eye movements. , How omnipause neurons function to gate the transition between fixation and saccadic eye movements by monosynaptically suppressing activity in premotor burst neurons during fixation and releasing them during saccades is shown schematically in Fig. 8.9 .
The control of microsaccades by premotor saccadic neurons
To explore the visual world, we make voluntary saccadic eye movements that are interspersed with periods of fixation. However, even when our gaze is fixed, our eyes are not completely still. Instead, we generate small fixational eye movements called microsaccades (for reviews, see , ). The direction and propensity of microsaccades can be biased by attention, but, on average, microsaccades are corrective such that the eyes remain focused on the target. , , Neural recordings in the saccadic pathway have established that the same premotor saccadic burst neurons that encode the size, duration, and velocity of saccades (i.e., Fig. 8.9 ), also encode these same measures during microsaccades. Furthermore, the same simple first-order model provides an adequate description of the relationship between neural responses and eye motion. Finally, omnipause neurons cease firing (pause) during microsaccades as they do during saccades , and show a comparable robust relationship between the pause duration and microsaccade duration. Thus, the saccadic burst generator controls both saccades and microsaccades.
Neural control of pursuit
Smooth pursuit eye movements are made to track a moving visual target of interest, so that its image is stabilized on the fovea to provide high visual acuity. Such eye movements are typically considerably slower than saccades, rarely reaching velocities beyond 100 degrees/s as compared with 500 to 700 degrees/s. The neural basis of smooth pursuit eye movements involves the transformation of visual target motion information—a sensory signal produced by target motion relative to retina—into the eye movement motor command required to stabilize the moving target on the fovea. This visuomotor transformation is largely accomplished via a corticopontocerebellar pathway (reviewed in , ) arising in areas of the extrastriate cortex and ending in the extraocular motoneurons ( Fig. 8.11 ).

The smooth pursuit system is generally modeled as a negative feedback controller in which a retinal velocity error signal (i.e., the difference between target velocity and eye velocity) drives eye movements with a 100 ms delay (reviewed in ). This is because the fixed delays inherent to the corticopontocerebellar pathway, which controls smooth pursuit eye movements, result in a around 100-ms latency between visual target motion input and the resultant pursuit eye movement. Importantly, this delay places a limit on tracking performance. In conditions where target velocity changes dynamically as a function of time, the current tracking eye velocity will always be around 100 ms behind the actual target velocity. For this reason, smooth pursuit performance becomes increasingly inaccurate for more dynamic targets, notably those moving at frequencies greater than about 1 Hz. In addition to retinal velocity error, retinal position and acceleration error signals can also drive pursuit eye movements. However, their relative influence is far less than that of retinal velocity error. Finally, nonvisual mechanisms such as anticipation and target predictability can improve pursuit performance. As detailed further, this integration of higher-order extraretinal signals already begins early in the corticopontocerebellar pathway generating smooth pursuit, at the level of cortex.
The visuomotor transformation that controls smooth pursuit eye movements can be understood through analysis of the differences in neuronal responses seen at each stage of this corticopontocerebellar pathway. First, neurons in middle temporal cortex (area MT) of extrastriate cortex encode sensory information, namely the direction and velocity of visual target motion relative to the retina. Area MT sends this information on to both the medial superior temporal cortex (area MST) and smooth eye movement region of the frontal eye fields (FEF SEM ), where visual sensory signals begin the transformation to eye motor commands. Notably, area MST and FEF SEM neurons integrate extraretinal signals such as eye movement and predictive/anticipatory information with retinal sensory information to compute an estimate of the pursuit command required by downstream structures. These cortical areas then primarily transmit such information to the pursuit-related regions of the cerebellum via the dorsolateral pontine nucleus (dLPN).
The floccular lobe (flocculus and ventral paraflocculus) and oculomotor vermis comprise the pursuit-related regions of the cerebellum. These areas drive eye movements via their direct projections to premotor neurons in the brainstem. Specifically, cerebellar Purkinje cells in these regions of the cerebellum send inhibitory projections to target neurons located within the vestibular nuclei. These vestibular nuclei neurons, which are known as eye-head (EH) neurons, in turn directly project to the extraocular motoneurons located in the III, IV, and VI cranial nuclei to drive pursuit eye movements. Whereas EH neurons provide the most significant input to drive the motoneurons during smooth pursuit eye movements, neurons in other premotor brainstem nuclei, including the nucleus prepositus, also show clear modulation , , , and thus also contribute to generation of smooth pursuit eye movements.
Neurons at the final stages of the smooth pursuit pathway—both cerebellar Purkinje cells and EH premotor neurons—predominately encode motor signals. This finding indicates that visual sensory information (i.e., target motion relative to the retina) is successfully transformed into the required eye motor command by the smooth pursuit corticopontocerebellar pathway. Moreover, the pursuit regions of the cerebellum not only serve as an essential node of the visuomotor transformation required for pursuit eye movements, but also as a key site for guiding motor learning. Such cerebellar-dependent learning continuously calibrates that the eye movement motor commands relative to target motion to ensure that smooth pursuit remains accurate across our life span.
The neural control of voluntary binocular eye movements
Vergence eye movements are voluntarily made by foveated animals to redirect the gaze of both eyes between targets located at different distance (i.e., near versus far targets, or vice-versa). Notably, to precisely align the binocular gaze on a target located at a new distance, the two eyes must rotate by different amounts. The difference in the angles through which each of the two eyes rotate is termed the vergence eye movement. Specifically, any binocular eye movement can be described in terms of its vergence (vergence = left eye − right eye) versus conjugate (conjugate = [left eye + right eye]/2) components, where the left eye and right eye inputs in these computations can be either position or velocity signals. There are two types of voluntary vergence eye movements, which are each generated by distinct neural circuits. First, smooth vergence eye movements are relatively slow movements that are made to track and then fixate a target moving in depth. Second, disjunctive saccades are made to redirect our eye between targets in depth and comprise a vergence component that is much faster than that generated during smooth vergence. Additionally, there is also a type of reflexive vergence eye movement, termed accommodation vergence, which automatically generates convergence eye movements in response to changes in the refractive strength of the eye’s lens to ensure proper binocular alignment (see Chapter 3 ). The two distinct premotor pathways that control voluntary smooth vergence versus disjunctive saccadic eye movements are described in detail next.
The neural control of voluntary smooth vergence
When gaze redirection between near and far targets is accomplished without a saccade, it is referred to as smooth vergence. In the laboratory, smooth vergence eye movements can be studied in conditions in which the gaze is redirected to track an object moving along the midsagittal plane. In this condition, each eye rotates by the same angle but in opposite directions, resulting in a symmetric vergence eye movement ( Fig. 8.12A ). As each eye rotates equally but in opposite directions, the eye movement has only a vergence but no conjugate component. The maximum smooth vergence velocities achieved in such conditions are typically less than 50 degrees/s (reviewed in ). Thus, smooth vergence eye movements are more sluggish than saccades. These voluntary smooth vergence movements can be further divided into two subclasses: fast fusional vergence, for example, the eye movements made to track a target moving in depth (consider a tennis player tracking a tennis ball), and slow fusional vergence, for example, the eye movements made to maintain fused binocular vision on a near or far near target, which can be impaired in subjects with convergence insufficiency.
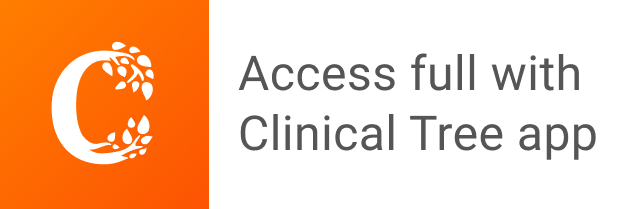