Introduction
Three fundamental visual sensory-motor tasks
The neural control of eye movements is organized to optimize performance of three general perceptual tasks. One task is to resolve the visual field while we move either by translation or rotation through space (self motion). Our body motion causes the image of the visual field to flow across the retina and reflexive eye movements reduce or stabilize this image motion to improve visual performance. The second task is to resolve objects whose position or motion is independent of the background field (object motion). Eye movements improve visual resolution of individual objects by maintaining alignment of the two foveas with both stationary and moving targets over a broad range of directions and distances of gaze. The third task is to explore space and shift attention from one target location to another. Rapid eye movements place corresponding images on the two foveas as we shift gaze between targets lying in different directions and distances of gaze.
Three components of eye rotation
All three perceptual tasks require three-dimensional control of eye position. These dimensions are controlled by separate neural systems. As described Chapter 8 , three pairs of extraocular muscles provide control of horizontal, vertical, and torsional position of each eye. Eye movements are described as rotations about three principal axes as illustrated in Figure 9.1 . Horizontal rotation occurs about the vertical Z-axis, vertical rotation about the horizontal X-axis, and torsion about the line of sight or Y-axis. As described in Chapter 8 , the amount of rotation about each of the three principal axes that is needed to describe a certain direction of gaze and torsional orientation of the eye depends upon the order of sequential rotations (e.g. horizontal, followed by vertical and then torsional). Some oculomotor tasks, such as retinal image stabilization, utilize all three degrees of freedom whereas other tasks, such as voluntary gaze shifts, only require two degrees of freedom, i.e. gaze direction and eccentricity from primary position. As described by Donder’s law, torsional orientation of the eye is determined by horizontal and vertical components of eye position. Ocular torsion is independent of the path taken by the eye to reach a given eye position and is constrained by the gaze direction. Listing’s law quantifies the amount of ocular torsion at any given eye position, relative to the torsion of the eye in primary position of gaze.

Binocular constraints on eye position control
Binocular alignment of retinal images with corresponding retinal points places additional constraints on the oculomotor system. Because the two eyes view the world from slightly different vantage points, the retinal image locations of points subtended by near objects differ slightly in the two eyes. This disparity can be described with three degrees of freedom (horizontal, vertical, and torsional components) that are analogous to the angular rotations of the eye shown in Figure 9.1 . The main task of binocular eye alignment is to minimize horizontal, vertical, and cyclodisparities subtended by near targets on the two foveas. This requires a conjugate system that rotates the two eyes in the same direction and amount, and a disconjugate system that rotates the visual axes in opposite directions. As described by Hering, a common gaze direction for the two eyes is achieved by a combination of conjugate and disconjugate movements that are controlled by separate systems. The version system controls conjugate movements and the vergence system controls disconjugate movements.
Pure version and vergence movements are described respectively by the isovergence and isoversion contours shown in Figure 9.2 . The isovergence circle describes the locus of points that stimulate the same vergence angle in all directions of gaze. A different isovergence circle exists at each viewing distance. The isoversion lines describe the locus of points that stimulate the same version angle over a range of viewing distances in a common direction of gaze relative to the head. Pure vergence movements occur along any of the isoversion lines and not just along the central or midsagittal plane. Fixation changes along any other contour result from a combination of version and vergence movements. Both version and vergence movements are described as combinations of horizontal, vertical, and torsional rotations. For example there can be horizontal and vertical version and vergence movements. Torsional rotations are usually referred to as cyclorotations (e.g. cycloversion or cyclovergence). Hering’s law implies that there is equal innervation of yoked muscle pairs: “one and the same impulse of will directs both eyes simultaneously as one can direct a pair of horses with single reins.” The law should not be taken literally, because common gaze commands from higher levels are eventually parceled into separate innervation sources in the brainstem that control individual muscles in the two eyes.

Feedback and feedforward control systems
The oculomotor system requires feedback to optimize sensory stimuli for vision with a sufficiently high degree of precision. Feedback provides information about motor response errors based upon their sensory consequences, such as unwanted retinal image motion or displacement. This visual error information usually arrives too late to affect the current movement, because the time delays in the visual system are about 50–100 msec. Instead, it is used to adaptively adjust motor responses to minimize subsequent errors. Oculomotor systems use sensory information to guide eye movements in two different ways. Motor responses can be guided in a closed-loop mode with an ongoing feedback signal that indicates the difference between the desired and actual motor response, or they can operate without concurrent feedback in an open-loop mode. The closed-loop feedback mode is used to reduce internal system errors or external perturbations. A physical example of a closed-loop system is the thermostatic regulation of room temperature, e.g. if the outside temperature drops, the furnace will turn on so that the room temperature stays constant. Motor responses can also be controlled in an open-loop mode, without a concurrent feedback signal. A physical example of an open-loop system is a water faucet, e.g. if the pressure drops, the flow of water will also drop, because the valve does not compensate for the pressure drop.
The mode of the response depends on the latency of the response, its duration and velocity. In most examples, visual feedback in a closed-loop system is used to maintain or regulate a fixed position or slow movements of the eyes when there is adequate time to process the error signal. Errors in eye posture or movement are sensed from displacement of the object of regard from the fovea or slippage of the retinal image and negative feedback control mechanisms attempt to reduce the errors to zero during the response.
Feed-forward control systems do not utilize concurrent visual feedback and are described as open-loop. These systems can respond to non-visual (extra-retinal) stimuli, or they respond to advanced visual information with short latencies and brief durations. For example, brief rapid head movements stimulate vestibular signals that evoke compensatory eye movements to stabilize the retinal image. These head movements can produce retinal image velocities of 300–400 deg/sec, yet the eyes respond with a counter rotation within 14 msec of the movement. The oculomotor response to head motion must rely on vestibular signals since retinal image velocities produced by head rotation exceed the upper velocity limit for sensing motion by the human eye. Retinal image velocities that exceed this upper limit appear as blurred streaks rather than as moving images. Visual feedback is not available when the response to head rotation begins because the latency is too short to utilize concurrent visual feedback. A minimum of 50 msec is needed to activate cortical areas that initiate ocular following, such that any motor response with a shorter latency must occur without concurrent visual feedback . Some open-loop systems, such as brief rapid gaze shifts (saccades), respond to visual information sensed prior to the movement rather than during the movement. Their response is too brief to be guided by negative visual feedback. Accuracy of a feedforward system is evaluated after the response is completed. Visually sensed post-task errors are used by feedforward systems to improve the accuracy of subsequent open-loop responses in an adaptive process that calibrates motor responses. Calibration minimizes motor errors in systems that do not use visual feedback during their response. All feed-forward oculomotor systems are calibrated by adaptation and this plasticity persists throughout life.
Hierarchy of oculomotor control
The following sections present a functional classification of eye position and movement control systems used to facilitate three general perceptual tasks and a hierarchial description of their neuro-anatomical organization ( Box 9.1 ). A hierarchy of neural control exists within each of the functional categories of eye movements that plans, coordinates, and executes motor activity. Three pairs of extraocular muscles that rotate each eye about its center of rotation are at the bottom of this hierarchy. The forces applied by these muscle pairs to the eye are controlled at the level above by the motor nuclei of cranial nerves III, IV, and VI. Motor neurons in these nuclei make up the final common pathway for all classes of eye movements. Axon projections from these neurons convey information to the extraocular muscles for executing both slow and fast eye movements. Above this level, premotor nuclei in the brainstem coordinate the combined actions of several muscles to execute horizontal, vertical, and torsional eye rotations. These gaze centers orchestrate the direction, amplitude, velocity, and duration of eye movements. Interneurons from the premotor nuclei all converge on motor nuclei in the final common pathway. Premotor neurons receive instructions from supranuclear regions including the superior colliculus, the substantia nigra, the cerebellum, frontal cortical regions including the frontal eye fields (FEF) and supplementary eye fields (SEF), and extrastriate regions including the medial temporal visual area (MT), the medial superior temporal visual area (MST), the lateral intraparietal area (LIP), and the posterior parietal area (PP). These higher centers plan the desired direction and distance of binocular gaze in 3-D space. Cortical–spatial maps of visual stimuli are transformed into temporal codes for motor commands between cerebral cortex and ocular motoneurons, to which the superior colliculus and cerebellum contribute. They determine when and how fast to move the eyes to fixate selected targets in a natural complex scene or to return them to a remembered gaze location. The following sections will discuss the hierarchical control for each of three functional classes of eye movements. The next section describes the final common pathway that conveys innervation for all classes of eye movements.
Subcortical oculomotor disorders are classified by lesion sites in the hierarchy of motor control:
- •
Peripheral (cranial nerves and muscles)
- •
Nuclear (cranial motor nuclei making up the final common pathway)
- •
Premotor (coordinates combined actions of several muscles)
- •
Internuclear (connections between nuclear and premotor sites)
- •
Supranuclear (motor planning stage)
Final common pathway
Cranial nerves: III, IV, & VI and motor nuclei
Cranial nerves III, IV, and VI represent the final common pathway as defined by Sherrington, for all classes of eye movements. All axon projections from these cranial nuclei carry information for voluntary and reflex fast and slow categories of eye movements. The oculomotor (III), trochlear (IV), and abducens (VI) nuclei innervate the six extraocular muscles, iris and ciliary body. The abducens nucleus innervates the ipsilateral lateral rectus. Premotor interneurons also project from VI to the contralateral oculomotor nucleus for control of the contralateral medial rectus, to produce yoked movements on lateral gaze that are consistent with Hering’s law. The trochlear nucleus innervates the contralateral superior oblique. The oculomotor nucleus innervates the ipsilateral medial rectus, inferior rectus, and inferior oblique, and the contralateral superior rectus. The anterior portion of the oculomotor nucleus also contains motor neurons that control pupil size and accommodation in a specialized region called the Edinger–Westphal nucleus. Afferents from this nucleus synapse in the ciliary ganglion prior to innervating their target muscles. The regions of the oculomotor nucleus that control various eye muscles are illustrated in Figure 9.3 .

Motor neuron response
The motor neurons control both the position and velocity of the eye. They receive inputs from burst and tonic cells in premotor nuclei. The tonic inputs are responsible for holding the eyes steady, and the more phasic or burst-like inputs are responsible for initiating all eye movements to overcome orbital viscosity and for controlling eye movements. All motor neurons have the following characteristics as illustrated in Figure 9.4 .
- 1
They have on–off directions (they increase their firing rate in the direction of agonist activity).
- 2
All cells participate in all classes of eye movements including steady fixation.
- 3
Each cell (especially tonic) has an eye position threshold at which it begins to fire. Motor neurons have thresholds that range from low to high. Cells with low thresholds begin firing when the eye is in the off field of the muscle that it innervates. Cells with higher thresholds can begin to fire after the eye has moved past the primary position by as much as 10 degrees into the on field of the muscle. The graded thresholds of motor neurons are responsible for the recruitment of active cells as the eyes move into the field of action for the muscle.
- 4
Increasing the frequency of spike potentials for a given neuron increases contractile force. Once their threshold is exceeded all cells increase their firing rate as the eye moves further along in the on direction of the muscle until they saturate. Cells increase their firing rate linearly as the eye moves into their on field.

Functional classification into three general categories
I
Stabilization of gaze relative to the external world
Movements of the head during locomotion tasks such as walking are described by a combination of angular rotations and linear translations. The oculomotor system keeps gaze fixed in space during these head movements by using extra-retinal and retinal velocity information about head motion. The primary extra-retinal signal comes from accelerometers in the vestibular apparatus.
A
Extra-retinal signals
The vestibular system contains two types of organs that transduce angular and linear acceleration of the head into velocity signals ( Fig. 9.5 ). , Three semicircular canals lie on each side of the head in three orthogonal planes that are approximately parallel to a mirror image set of planes on the contralateral side of the head. These canals are stimulated by brief angular rotations of the head and the resulting reflexive ocular rotation is referred to as the vestibulo-ocular reflex (angular VOR). In addition, two otoliths (the utricle and sacculus) transduce linear acceleration caused by head translation as well as head pitch (tilt about the interaural axis) and roll (tilt about the nasal–occipital axis) into translation velocity and head orientation signals (linear VOR). Angular acceleration signals stimulate the semicircular canals and result in eye rotations that are approximately equal and opposite to the motion of the head. This stabilization reflex has a short 7–15-msec latency because it is mediated by only three synapses and is accurate for head turns at velocities in excess of 300 deg/s. Hair cells in the canals can be stimulated by irrigation of one ear with cold water. This produces a caloric-vestibular nystagmus that causes the eyes to rotate slowly to the side of the irrigated ear. These slow-phase movements are interrupted by fast saccadic eye movements that reset eye position in the reverse direction (fast phase). A sequence of slow and fast phases is referred to as jerk nystagmus ( Fig. 9.6 ). Head rotations about horizontal, vertical, and nasal–occipital axes produce VOR responses with horizontal, vertical, and torsional counter-rotations of the slow phase of the nystagmus.


To be effective, these reflex eye rotations must stabilize the retinal image. If the axis of angular head rotation coincided with the center of eye rotation, perfect compensation would occur if angular eye velocity equaled angular head velocity. However, the axis of head rotation is the neck and not the center of eye rotation such that when the head rotates, the eyes both rotate and translate with respect to the visual field. This is exacerbated during near viewing conditions. To stabilize the retinal image motion of a nearby target caused by angular head movements, the eye must rotate more than the head. Indeed, the gain of the VOR increases with convergence. Mismatches between eye and head velocity also occur with prescription spectacles that magnify or minify retinal image motion. Because the VOR responds directly to vestibular and not visual stimuli, its response is classified as open loop. The VOR compensates for visual errors by adapting its gain in response to retinal image slip to produce a stabilized retinal image. Perfect compensation would occur if angular eye velocity were equal and opposite to angular head velocity while viewing a distant scene. However empirical measures show that at high oscillation frequencies (2 Hz) compensation by the VOR is far from perfect and yet the world appears stable and single during rapid head shaking without any perceptual instability or oscillopsis. Thus, to perceive a stable world, the visual system must be aware of both the amount of head rotation and the inaccuracy of compensatory eye movements so that it can anticipate any residual retinal image motion during the head rotation.
Linear acceleration signals and gravity stimulate the otoliths and result in ocular rotations that are approximately 10 percent of the static head tilt caused by pitch and roll from vertical. Head pitch causes vertical eye rotations and head roll causes ocular torsion in the direction opposite to the head roll, “the ocular counterrolling reflex”. , Head roll can also elicit skew movements. When the otolith membranes are displaced on their maculae by inertial forces during rapid linear acceleration, such as during takeoff of an aircraft, a false sense of body pitch called the somatogravic illusion can occur. This illusion results from perceiving vertical as the non-vertical combination of gravitational and acceleration-inertial force vectors. The non-vertical gravitoinertial force causes pilots to perceive the aircraft as pitched upward during takeoff, and if the pilot corrects the false climb this can cause the aircraft nose to pitch downward with dangerous consequences.
Disorders of the vestibular system can produce imbalanced otolith inputs that can result in large amounts of vertical divergence of the eyes (skew deviation) by as much as 7 degrees, bilateral conjugate ocular torsion by as much as 25 degrees, and paradoxical head tilt known as the ocular tilt response (OTR). For example, if the head is tilted to the left, there is paradoxical torsion of both eyes in the direction of head roll and the left eye is rotated downward with respect to the right eye. Manifestations of the OTR will be discussed in the section on nystagmus.
B
Retinal signals
Head motion also produces whole-field retinal image motion of the visual field (optic flow). These retinal signals stimulate reflexive compensatory eye rotations that stabilize the retinal image during slow or long-lasting head movements. The eyes follow the moving field with a slow phase that is interrupted by resetting saccades (fast phase) 1 to 3 times per second. This jerk nystagmus is referred to as optokinetic nystagmus (OKN) and it complements the VOR by responding to low-velocity sustained head movements such as those that occur during walking and posture instability. Like the VOR, OKN also responds with horizontal, vertical and cyclo eye rotations to optic flow about vertical, horizontal, and nasal–occipital axes.
The optokinetic response to large fields has two components, including an early and delayed segment (OKNe and OKNd). OKNe is a short-latency ocular following response (<50 msec) that constitutes the rapid component of OKN, and OKNd builds up slowly after 7 seconds of stimulation. OKNe is likely to be mediated by the pursuit pathway. The delayed component is revealed by the continuation of OKNd in darkness (optokinetic after nystagmus, OKAN). OKNd results from a velocity memory or storage mechanism. , The time constant of the development of the OKAN matches the time constant of decay of the cupula in the semicircular canals. Thus OKAN builds up so that vision can compensate for loss of the vestibular inputs during prolonged angular rotation that might occur in a circular flight path. OKN can be used clinically to evaluate visual acuity objectively by measuring the smallest texture size and separation in a moving field that elicits the reflex.
C
Neuro-control of stabilization reflexes
1
Vestibulo-ocular reflex
The transducer that converts head rotation into a neural code for driving the VOR consists of a set of three semicircular canals paired on each side of the head. The horizontal canals are paired and the anterior canal on one side is paired with the posterior canal on the contralateral side ( Fig. 9.5 ). These are opponent pairs so that when one canal is stimulated by a given head rotation its paired member on the contralateral side is inhibited. For example, downward and forward head motion to the left causes increased firing of the vestibular nerve for the left anterior canal and decreased firing of the vestibular nerve projections from the right posterior canal. The three canals lie roughly in the pulling directions of the three muscle planes. Thus the left anterior canal and right posterior canal are parallel to the muscle planes of the left eye vertical recti and the right eye obliques. Pathways for the horizontal VOR are illustrated in Figure 9.7 for a leftward head rotation. Excitatory innervation projects from the left medial vestibular nucleus to the right abducens nucleus to activate the right lateral rectus, and an interneuron from the right abducens nucleus projects to the left oculomotor nucleus to activate the left medial rectus. The abducens serves as a premotor nucleus to coordinate conjugate horizontal movements to the ipsilateral side in accordance with Hering’s law.

The cerebellar flocculus is essential for adaptation of the VOR to optical distortions such as magnification. The flocculus receives excitatory inputs from retinal image motion (retinal slip) and head velocity information (canal signals) and inhibitory inputs from neural correlates of eye movements that provide a negative feedback signal. Adaptation only occurs if retinal image motion and head turns occur together. The gain of the VOR will be adapted to decrease whenever retinal slip and head turns are in the same direction, and to increase whenever they are in opposite directions. Following adaptation, an error correction signal is projected from the flocculus by Purkinje cells to floccular target neurons (FTN) in the vestibular nucleus to make appropriate changes in VOR gain.
2
Optokinetic nystagmus
The visual stimulus for OKN is derived from optic flow of the retinal image. The retina contains ganglion cells that respond exclusively to motion in certain directions or orientations. This information passes along the optic nerve, decussates at the chiasm and projects to the cortex via the geniculate body (LGN) or to the midbrain via the accessory optic tract ( Fig. 9.8 ). This tract has several nuclei in the pretectal area. One pair of these nuclei, the nucleus of the optic tract (NOT), is tuned to horizontal target motion to the ipsilateral side (i.e. nasal to temporal motion). The lateral and medial terminal nuclei (LTN and MTN) are tuned for vertical target motion. Neurons in these nuclei only receive subcortical inputs from the contralateral eye. They have large receptive fields and respond to large textured stimuli moving in specific directions. Stimulation of the right NOT with rightward motion causes following movements of both eyes to the right or ipsilateral side, and similarly stimulation of the left NOT with leftward motion causes leftward conjugate following movements. Each NOT projects signals via the inferior olive to the vestibular nuclei and possibly to the flocculus via the climbing fibers of the cerebellum. The NOT provides a visual signal to the vestibular nucleus, and the motor response is the same as for velocity signals originating from the semicircular canals.

The cortical region that organizes motion signals is the medial superior temporal lobe (MST). This region is important for generating motion signals for both pursuit and OKN. Binocular cortical cells receive projections from both eyes and code ipsilateral motion from the contralateral visual field at higher velocities than the subcortical system. The cortical cells project to the ipsilateral NOT.
Until the age of 3–4 months, the monocular subcortical projections predominate because the cortical projection has not yet developed. As a result, OKN in infancy is driven mainly by the crossed subcortical input. The consequence is that monocular stimulation only evokes OKN with temporal-to-nasal motion but not with nasal-to-temporal motion. After 3–4 months of development, the infant’s cortical projections predominate and horizontal OKN responds to both monocular temporal-ward and nasal-ward image motion. The cortical projections to the NOT fail to develop in infantile esotropia and as adults these patients exhibit the same asymmetric OKN pattern as observed in immature infants This anomalous projection is responsible for a disorder known as latent nystagmus in which a jerk nystagmus occurs when one eye is occluded with the slow phase directed toward the side of the covered eye. During monocular fixation, the stimulated retina increases the activity of neurons in the contralateral NOT via subcortical crossed projections, but it is unable to innervate the ipsilateral NOT via the ineffective cortical-tectal projection. The result is that both eyes’ positions are drawn to the side of the stimulated NOT (i.e. the side of the covered eye). The fixation error is corrected with a saccade and a repeated sequence is described as latent or occlusion nystagmus.
II
Foveal gaze lock (maintenance of foveal alignment with stationary and slowly moving targets)
A
Static control of eye alignment (fixation)
The oculomotor system enhances visual resolution by maintaining alignment of the fovea with attended stationary and moving targets. , During fixation of stationary targets, the eyes sustain foveal alignment over a wide range of target locations in the visual field. Gaze direction is controlled by a combination of eye position in the orbit and head position. Gaze is mainly controlled by eye position for targets lying at eccentricities of less than 15 degrees from primary position. Steady fixation at larger gaze eccentricities is accomplished with a combination of head and eye position. Holding eye fixation at large eccentricities (>30 degrees) without head movements is difficult to sustain and the eye drifts intermittently toward primary position in gaze evoked nystagmus. This drift is exacerbated by alcohol. Even within the 15-degree range, the fixating eye is not completely stationary. It exhibits physiological nystagmus that is composed of slow horizontal, vertical, and torsional drifts (0.1 deg/sec), micro-saccades (<0.25 deg), and a small amplitude (<0.01 deg) high frequency tremor (40–80 Hz). Some of the drifts and saccades are error producing, while others are error correcting when they serve to continually minimize motion and adjust the alignment of the target of regard with the fovea. , The high frequency tremor reflects the noise possibly originating from asynchronous firing of individual motoneurons that is filtered by the mechanical properties of the eye. Gaze evoked nystagmus can be associated with impaired smooth pursuit and paramedian pontine lesions.
Stereoscopic depth perception is enhanced by accurate binocular fixation. , Precise bifoveal alignment requires that the eyes maintain accurate convergence at the distance of the attended target. Small constant errors of convergence during attempted binocular fixation (<15 arc min) are referred to as fixation disparity, and these can impair stereo performance. Fixation disparity is a closed-loop error because it occurs in the presence of retinal image feedback from binocular disparity. Fixation disparity results from incomplete nullification of an open-loop error of convergence known as heterophoria. Heterophoria equals the difference between the convergence stimulus and the convergence response measured under open-loop conditions (e.g. monocular occlusion). The magnitude of fixation disparity increases monotonically with the disparity stimulus to convergence when it is varied with horizontal prisms. The slope of this function is referred to as the forced-duction fixation disparity curve. Shallow slopes of these curves indicate that the heterophoria is reduced by adaptation during binocular or closed-loop conditions. Vergence adaptation is very rapid. For example, when convergence is stimulated for only one minute with a convergent disparity and then one eye is occluded, the convergence response persists in the open-loop state. Typically the convergent disparity is produced by prisms that deflect the perceived direction of both eye’s images inward, or in the nasal-ward direction (i.e. the base of the prism before each eye is temporal-ward or “base-out”). This adapted change in heterophoria is constant in all directions of gaze and is referred to as concomitant adaptation. Prism adaptation is in response to efforts by closed-loop disparity vergence to nullify the open-loop vergence error (heterophoria). Prism adaptation improves the accuracy of binocular alignment and stereo-depth performance.
Horizontal vergence equals the horizontal component of the angle formed by the intersection of the two visual axes and it is described with three different units of measurement or scales that include the degree, prism diopter (PD), and meter angle (MA). Prism diopters equal 100 times the tangent of the angle. Meter angles equal the reciprocal of the viewing distance (specified in meters) at which the visual axes intersect as measured from the center of eye rotation. A target at one meter stimulates one meter angle of convergence (approximately 3.4 degrees) and one diopter of accommodation. Prism diopters can be computed from meter angles by the product of MA and the interpupillary distance (IPD), measured in cm. For example, convergence at a viewing distance of 50 cm by two eyes with a 6 cm IPD equals either 2 MA or 12 PD. The advantage to units in MA is that the magnitude of the stimulus to accommodation in diopters is approximately equal to the magnitude of the stimulus to convergence, assuming that both are measured from a common point such as the center of eye rotation. This assumption produces large errors for viewing distances less than 20 cm because accommodation is usually measured in reference to the corneal apex that is 13 mm anterior to the center of eye rotation. The advantage to units in prism diopters is that they are easily computed from the product of MA and IPD. The advantage to units in degrees is that they accurately quantify asymmetric convergence where targets lie at different distances from the two eyes.
The Maddox classification describes open- and closed-loop components that make up the horizontal vergence response. The classification includes three open-loop components that influence heterophoria. These include an adaptable intrinsic bias (tonic vergence), a horizontal vergence response to monocular depth cues (proximal vergence), and a horizontal vergence response that is coupled with accommodation by a neurological cross-link known as accommodative-convergence. Tonic vergence has an innate divergence posture (5 degrees) known as the anatomical position of rest. Tonic vergence adapts rapidly to compensate for vergence errors during the first 6 weeks of life. At birth the eyes diverge during sleep, but after 6 weeks the visual axes are nearly parallel during sleep. The adapted vergence, measured in an alert state, in the absence of binocular stimulation and accommodative effort, is referred to as the physiological position of rest. The physiological position of rest equals the sum of tonic vergence and the anatomical position of rest. Tonic vergence adaptation ability continues throughout life to compensate for trauma, disease, and optical distortions from spectacles and aging factors.
Proximal vergence describes the open-loop vergence response to distance percepts stimulated by monocular depth cues such as size, overlap, linear perspective, texture gradients, and motion parallax. , This proximal response accounts for a large portion of the convergence response to changing distance. , The open-loop convergence response is also increased by efforts of accommodation. , The eyes maintain approximately 2/3 of a meter angle of convergence (4 prism diopters) for every diopter of accommodation. A target at one meter stimulates one meter angle of convergence (approximately 3.4 degrees) and one diopter of accommodation. Thus when the eyes accommodate 1 diopter the accommodative-convergence response increases by approximately 2.3 degrees or 4 prism diopters which is only 68 percent of the convergence stimulus. The sum of the three open-loop components of convergence typically lags behind the convergence stimulus and produces an open-loop divergence error (exophoria) that is usually less than 2 degrees under far viewing conditions and 4 degrees at near viewing distances. Esophoria describes open-loop vergence errors caused by excessive convergence that lead the stimulus. The distribution of heterophoria in the general population is not Gaussian or normally distributed. It is narrowly peaked about a mean close to zero, indicating that binocular errors of eye alignment are minimized by an adaptive calibration process. During closed-loop stimulation, vergence error is reduced to less than 0.1 degree by the component of the Maddox classification controlled by visual feedback, disparity vergence, which is stimulated by retinal image disparity. Variation in any of the three open-loop components of convergence will influence the magnitude of heterophoria and the resulting closed-loop fixation disparity. Fixation disparity acts as a stimulus to maintain activity of the disparity vergence so that it continues to sustain its nulling response of the underlying heterophoria during attempted steady binocular fixation. Other dimensions of vergence also adapt to disparity stimuli. Vertical vergence adapts to vertical prism before one eye, and cyclovergence adapts to optically produced cyclodisparity stimuli.
B
Dynamic control of eye alignment (smooth tracking responses to open- and closed-loop stimuli)
1
Conjugate smooth pursuit tracking
Smooth following pursuit movements allow the eyes to maintain foveal alignment with a moving target that is voluntarily selected. Pursuit is defined as the conjugate component of smooth following eye movement responses to target motion. As described below, disconjugate following responses to changes in target distance are referred to as smooth vergence. Conjugate pursuit differs from the delay portion of OKN, which is a reflex response to optic flow of the entire visual field. A conflict occurs between the pursuit and optokinetic systems when the eyes pursue an object that is moving across a stationary background. Pursuit stabilizes the moving target on or near the fovea but it causes optic flow or retinal slip of the stationary background scene. Conflicts can also occur between pursuit and the VOR if gaze is controlled with head tracking movements. Stabilizing the retinal image of a moving object with head movements produces a vestibular signal from the canals. Consequently, pursuit of a small moving target against a stationary background with eye or head movements requires that OKN and the VOR be ignored or suppressed. This is accomplished most effectively when the background lies at a different distance than the pursuit target.
Pursuit responds to target velocities ranging from several minutes of arc/sec to over 175 deg/sec. The gain or accuracy of pursuit is reduced as target velocity increases above 100 deg/sec. The VOR has a much higher velocity range than pursuit. This can be demonstrated by comparing two views of your index finger. Either keep your finger stationary while you shake your head rapidly from side to side at 2–3 Hz, or shake your finger at the same frequency without moving your head. The eye cannot follow the moving finger, but it can follow the stationary finger while shaking your head even though the head-relative motion is identical in these two examples. The pursuit response is more accurate when the target motion is predictable such as with pendular motion. Pursuit errors are reduced by modifying pursuit velocity and with small catch-up saccades. The combination of pursuit and catch-up saccades that appear at low stimulus velocities in patients with pursuit deficits is referred to as cogwheel pursuit. The ratio of eye velocity over target velocity (gain) or accuracy of pursuit is normally affected by target visibility (contrast) as well as drugs and fatigue. ,
The pursuit response to sudden changes in target velocity has a short latency (80–130 msec), and is composed of two general phases referred to as open-loop and closed-loop ( Fig 9.9 ). Pursuit is initiated during the open-loop phase and it is maintained during the closed-loop phase. The open-loop response is divided into an early and late component. The early component is a feed forward phase that lasts for only 20 msec. During this early phase, there is a rapid acceleration of the eye (40–100 deg/sec/sec) that is in the correct direction but is independent of the stimulus velocity and initial retinal image position. During the late open-loop component that lasts 80 msec, the initiation of pursuit depends strongly on target velocity and retinal image position. Eye acceleration is highest in response to targets imaged near the fovea and decreases sharply with increasing eccentricity up to 21 degrees. These open-loop components are calibrated by adaptation.

Pursuit is maintained during the closed-loop phase in response to negative feedback from retinal image velocity (retinal slip) and position, as well as an internal estimate of target velocity relative to the head. If the eye lags behind the stimulus, the retinal image velocity is not nulled leaving a residual retinal image slip and position error away from the fovea. The pursuit system accelerates to correct both retinal position and velocity errors. When pursuit is very accurate and there is no retinal error, the eye continues to pursue the target without the eye-referenced signals. Pursuit is maintained by an internal estimate of target velocity or a head referenced motion signal that is computed from a combination of retinal slip and an internal representation of eye velocity. This can be demonstrated by attempting to fixate an ocular floater or a retinal afterimage that is located near the fovea. Attempts to foveate the stabilized retinal image lead to smooth following eye movements even though the retinal image is always motionless. The eye is tracking an internal correlate of its own motion that causes the target to appear to move with respect to the head. Cognitive factors including attention, prediction, and learning are able to influence the execution of smooth pursuit.
2
Disconjugate smooth vergence tracking
Foveal alignment of targets that move slowly in depth is maintained by smooth vergence following eye movements. , In addition to improving stereoscopic depth perception, smooth vergence provides information about changing target distance that affects size and depth perception. Slow changes in smooth vergence respond to body sway and posture instability. While smooth vergence responses can be very inaccurate during natural rapid head movements, when the head is stationary they are very accurate at temporal frequencies up to 1 Hz. At higher frequencies accuracy is reduced but it can improve with small disparity stimuli. It is likely that accuracy of smooth vergence is task dependent. Smooth vergence accuracy is more demanding for spatial localization tasks that lack depth cues other than disparity, compared to tasks that have ample monocular cues for direction and distance. The accuracy of smooth vergence responses to changing disparity improves with predictable target motion. Stimuli for smooth vergence tracking include magnitude and velocity of retinal image disparity and perceptual cues to motion in depth, including size looming. , Smooth vergence tracking is very susceptible to fatigue and central nervous system suppressants.
3
Adaptable interactions between smooth pursuit and smooth vergence
In natural scenes, motion of a target in the fronto-parallel plane is tracked binocularly with conjugate smooth pursuit. However in conditions of anisometropia corrected with spectacle lenses, the image motion of the two eyes is magnified unequally and this produces variations of binocular disparity that increase with target eccentricity from the optical centers of the lenses. Tracking motion of targets in the fronto-parallel plane then requires both smooth pursuit and smooth vergence eye movements such that one eye moves more than the other does. The oculomotor system can adapt to the binocular disparity that changes predictably with eye position. Adaptation produces open-loop non-conjugate variations of heterophoria that compensate for the horizontal and vertical disparities produced by the unequal magnifiers during smooth vergence tracking responses. After only one hour of pursuit tracking experience with anisometropic spectacles, one eye can be occluded and the two eyes continue to move unequally during monocular tracking. The adapted heterophoria is coupled to vary with both eye position and direction of eye movement.
C
Neuro-control of smooth foveal tracking
1
Smooth pursuit tracking system
Smooth following eye movements result from cortical motion signals in extrastriate cortex in areas MT and MST that lie in the superior temporal sulcus. , Area MT encodes speed and direction of visual stimuli in three dimensions relative to the eye. MT receives inputs from the primary visual cortex and projects visual inputs to area MST and the frontal eye fields (FEF). Cells in MST fire in concert with head-centric target movement; i.e. they combine retinal and efference copy signals. Each hemisphere of the MST codes motion to the ipsilateral side. Cells have two types of visual motion sensitivity; they respond to motion of large-field patterns and small spots but the direction preferences for the two stimulus types are in opposite directions. The anti-directional large-field responses could facilitate pursuit of small targets moving against a far stationary field, and motion parallax stimuli. Efferents from MST and FEF project ipsilaterally to the NOT to generate OKN and to the dorsal lateral pontine premotor nuclei (DLPN) for pursuit tracking.
The DLPN plays a large role in maintaining steady-state smooth pursuit eye velocity, and the nucleus reticularis tegmenti pontis (NRTP) contributes to both the initiation and maintenance of smooth pursuit. Neurons in these areas are primarily encoding aspects of eye motion with secondary contributions from retinal signals such as those coded in the NOT. Velocity signals are projected from DLPN to the floccular region and to the vermis lobules VI and VII of the cerebellum. The DPN serves as a precerebellar relay for both pursuit and saccade-related information, and these two types of eye movements may represent different outcomes from a shared cascade of sensory-motor functions. The flocculus is thought to maintain pursuit eye movements during steady constant tracking while the vermis is important when the target velocity changes or when initiating pursuit. The role of the cerebellum is to sort out eye and head rotations in the tracking process and to sort out the ocular pursuit signal from visual and eye–head motor inputs. From here, activity passes via parts of the vestibular nuclei, which perform the necessary neural integration of the velocity signal to a position signal that is sent to the eye muscle motor neurons.
2
Smooth vergence tracking system
Vergence results from the combined activity of intrinsic tonic activity, accommodative vergence, and responses to binocular disparity and perceived distance. The sensory afferent signals for vergence (binocular disparity and blur) are coded in the primary visual cortex (area V1). Some cells in V1 incorporate vergence to code egocentric (head-referenced) distance. Cells in area MT and MST respond to retinal disparity and changing size. Cells in the parietal cortex respond to motion in depth. Efferent commands for vergence appear in cells in the frontal eye fields. In the midbrain the premotor NRTP, located just ventral to the rostral portion of the paramedian pontine reticular formation (PPRF), receives projections from the frontal eye fields and the superior colliculus. Lesions in this region result in deficits of slow-continuous and fast-step vergence control. , The NRTP projects to the cerebellum, and appears to be associated with vergence and accommodation. The dorsal vermis is involved in the conversion of 3D pursuit signals to control signals for vergence eye movements. , The posterior interposed nucleus (PIN) of the cerebellum projects to supraoculomotor regions that contain near response cells in the mesencephalic reticular formation (MRF). , The supraoculomotor nucleus contains both burst and tonic neurons. The burst cells code velocity signals for smooth vergence, and the tonic neurons code position signals to maintain static vergence angle. Excitatory connections of the supraoculomotor nucleus project to the oculomotor nucleus, driving the medial recti. Inhibitory connections project to the abducens nucleus to inhibit the lateral rectus. The supraoculomotor nucleus relays control of both accommodative and disparity vergence.
III
Foveal gaze shifts: target selection and foveal acquisition
A
Rapid conjugate shifts of gaze direction (saccadic eye movements)
Saccades are very fast, yoked eye movements that have a variety of functions. , They produce the quick phase of the VOR and OKN to avoid turning the eyes to their mechanical limits. They reflexively shift gaze in response to novel stimuli that appear unexpectedly away from the point of fixation. Saccades shift gaze during reading from one group of words to another. Saccades search novel scenes to assist us in acquiring information. They also return gaze to remembered spatial locations. Two primary functions in all of these tasks are to move the eye rapidly from one position to another and then maintain the new eye position. The rapid movement is controlled by a pulse and slide innervation pattern and the position is maintained by a step innervation.
The separate components of innervation for the saccade match the characteristics of the plant (i.e. the globe, muscles, fat, and suspensory tissues). The rapid changes in orbital position are made by the saccade at a cost of considerable energy. Saccade velocities can approach 1000 deg/sec. In order to achieve these high velocities a phasic level of torque is needed to overcome the viscosity of orbital tissues, most of which is in the muscles. The phasic-torque is generated by a large brief force resulting from a pulse or burst of innervation. The torque is dissipated or absorbed by the muscles, so that the force developed by the pulse of innervation does not reach the tendon (see Chapter 8 – Three-Dimensional Rotations of the Eye). At the end of the saccade, a lower constant force resulting from a step innervation generates a tonic level of torque that is needed to hold the eye still against the elastic restoring forces of the orbital tissues. The eye positions resulting from the pulse and step forces must be equal to produce rapid gaze shifts. Pulse-step mismatches will result in rapid and slow components of gaze shifts. For example, if the pulse is too small, the saccade will slide (post-saccadic drift, called a glissade) to the new eye position at the end of the rapid phase of the saccade. The slide component is adaptable as has been shown by long-term exposure to artificially imposed retinal image slip immediately after each saccade. The adapted post-saccadic drift cannot be explained by an adjustment of the pulse-step ratio, suggesting that the slide innervation is an independent third component of saccadic control (pulse-slide-step). Slide innervation produces a phasic-torque in addition to the pulse component that adjusts the duration and velocity of the saccade so that its amplitude matches the position maintained by the step component. The pulse, slide and step are all under independent, cerebellar control, with the primary goal of protecting vision by preventing retinal slip, and a secondary goal of making accurate saccades.
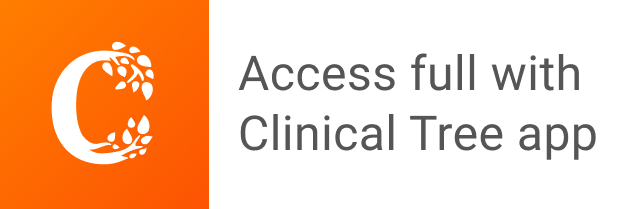