Vision from starlight to sunlight
The human visual system operates effectively over an enormously wide range of intensities, of at least a billion-fold, from around 10 −4 cd m −2 under starlight conditions to around 10 5 cd m −2 under intense sunlight. Changes in pupil area account for only about 1 log unit of this 9 log-unit range, since the pupil diameter changes from a maximum of 8 mm to a minimum of 2.5 mm, corresponding to about a 10-fold reduction in area. Instead, the great bulk of the operational range is achieved by the combination of, first, a switch between the scotopic (rod-based) and photopic (cone-based) pathways in our duplex visual system and, second, the ability of each of these photoreceptor systems to operate over a range of 5 log units (100,000-fold) or more.
Light adaptation versus dark adaptation
This ability of the visual system (or of any of its component parts, such as a photoreceptor) to adjust its performance to the ambient level of illumination is known as “light adaptation.” This adjustment typically occurs very rapidly (within seconds), whether the light intensity is increasing or decreasing. The term “dark adaptation” is reserved for the special case of recovery in darkness, following exposure of the eye to extremely bright and/or prolonged illumination that activates (and thereby bleaches) a substantial fraction of the visual pigment, rhodopsin, or its cone equivalent. Dark adaptation occurs slowly, and full recovery of the scotopic visual system after a very large bleach can take as much as an hour.
Light adaptation and the changes that accompany it are beneficial to the possessor of the eye. At very low intensities, visual sensitivity is increased to the utmost possible, so that the rod photoreceptors reliably signal the arrival of individual photons, and the scotopic visual system operates in a photon-counting mode. This ability of the scotopic system to operate at incredibly low intensities is enhanced by two deliberate trade-offs that permit more reliable detection of small signals in the presence of noise: first, reduced spatial resolution (i.e., increased spatial summation), and second, reduced time resolution (i.e., increased temporal integration). Similar trade-offs are used in the photopic system, so that as the ambient illumination decreases from daylight levels toward twilight levels, one’s spatial and temporal resolution deteriorate, making it very difficult to play fast ballgames when the light fades.
In contrast, the changes that characterize dark adaptation are disadvantageous. To be effectively blind to dim stimuli for some considerable time after intense light exposure, cannot in any way be useful to an organism. Indeed, for our ancestors (as well as ourselves) entering a cave from bright sunshine is likely to have been quite dangerous, given that visual sensitivity is greatly reduced for tens of minutes. Why should such an apparently unsatisfactory situation have persisted? One possibility is that this slowness of recovery represents an inevitable “cost” of the evolutionary changes that were needed to enable the scotopic system to signal individual photon hits. Another possible explanation is that a slow rod dark adaptation and the underlying slow rod visual pigment regeneration prevent excessive accumulation of light damage and oxidative stress to the rods during the day.
Purposes of light adaptation
A photoreceptor that did not adapt to the ambient light intensity would have a very narrow operating range of only 100-fold span of light responsiveness: low intensities would provide negligible response, whereas high intensities would saturate the cell. Accordingly, photoreceptor light adaptation can be viewed as a means for extending the operating range of the cell.
The purpose of light adaptation can be viewed more generally as being to permit the visual system (or any neuron within it) to provide the best possible performance at that particular level of illumination; however, in this context it is not always clear what constitutes “best”. For example, it is difficult for us to specify the time course of response that is best at any given level of illumination. A brief response is likely to provide a better reaction time for the organism, but it may result in very poor sensitivity; conversely, allowing the response to integrate for a longer period will provide greater sensitivity, but may result in a very poor reaction time.
In setting the best performance of a photoreceptor, one crucial task is to endow the cell with very high sensitivity at low light levels yet prevent it from saturating at higher light levels. Light adaptation accomplishes this by reducing the cell’s sensitivity to light as the background light level increases; however, this task needs to be performed in a manner that avoids excessive reduction in sensitivity. Cone photoreceptors excel in this capacity and are able to avoid saturation no matter how intense the steady light becomes, and to continue to signal contrast effectively. Rods, on the other hand, are only able to adjust their sensitivity over a relatively narrow range of intensities, before they are driven into saturation, thereby becoming completely unresponsive. One advantage of this saturation of the rods, however, is that it substantially reduces the metabolic demand of the rods for maintaining their transduction current and synaptic transmission at intensities at which the cones are already functional.
In addition to the very important function of optimizing the photoreceptor’s sensitivity over a broad range of light intensities, there are two other ways in which photoreceptor light adaptation optimizes the cell’s response. First, as will be explained below, when Eq. 20.1 is presented, Weber Law light adaptation permits automatic extraction of the contrast in the visual scene, independent of the absolute level of illumination. Second, light adaptation provides real-time adjustment of the time course of the response to an incremental flash of light in a manner that is presumed to be optimal for the visual system, although, as explained before, we are not yet able to quantify optimality in this regard.
Performance of the photopic and scotopic divisions of the visual system
Photopic vision: the cone system is the workhorse of vision
For humans, the photopic cone system can be considered the “workhorse” of vision because it is operational under almost all of the conditions that we experience (in the 21st century). Thus, it is the photopic system that underlies our sense of vision at all light levels apart from the exceptionally low intensities experienced in moonlit and starlit conditions. Under moonlight levels of illumination, our scotopic and photopic systems are both functional over an intensity range that is termed “mesopic.” To determine whether you are using your photopic system under twilight or nighttime conditions, there is a simple test: if you are able to detect any color in the scene, then your cones are active; if you cannot, then it is likely that only your rods are active. As the ambient intensity increases at dawn, the photopic system remains functional, up to the brightest sunlit conditions that we ever experience.
Despite their enormous importance to our vision, cones make up only about 5% of the population of photoreceptors. The low density of cone photoreceptors in the peripheral retina is quite adequate for our peripheral vision, even in daytime, as we require only relatively low spatial acuity in the periphery. Although the great majority of peripheral photoreceptors are rods, they are not in fact used under most of the circumstances that we think of as vision—instead, they are only used at exceedingly low ambient lighting levels. The reason for having a very high density of rods is to be able to capture every available photon under starlight conditions.
The responses of cones are rapid and moderately sensitive
One of the greatest advantages of cones over rods is their much faster speed of response. Our rods, even when they are light adapted, have responses that are much too slow to allow us to function visually at the speeds that are required to escape predators and to capture prey. Cones, instead, are specialized to permit extremely rapid signaling of visual stimuli to the brain.
Cones are often described as being far less sensitive than rods, but this view is misleading, especially when considered in terms of the rapidly changing visual stimuli that the cones are specialized for signaling. Although a cone may exhibit a peak sensitivity to a brief flash of light that is perhaps 30-fold lower than in a rod, the sensitivity to rapidly fluctuating stimuli is considerably higher in cones than in rods; thus, the slow response of the rod makes it very insensitive to rapidly changing stimuli. When calculated in terms of the efficacy of activation in the G-protein cascade of phototransduction, the “amplification” in cones and rods appears to be essentially indistinguishable. The observed difference in sensitivity measured at the peak of the response to a flash instead stems from a difference in the speed of response inactivation.
Comparison of photopic and scotopic light adaptation
A classical result comparing light adaptation in the photopic and scotopic divisions of the visual system is illustrated in Fig. 20.1A , from the work of Stiles. The threshold of a human subject for the detection of a flash is plotted against background intensity in double logarithmic coordinates. In this panel, the stimulus conditions provided a rod-based scotopic sensitivity that was only about 30× better than the cone-based photopic sensitivity—thus, the parafoveal region was tested with a small diameter yellow/green test flash (1 degree diameter, 60 ms, 580 nm) on a green background. The measurements are well described by Weber law curves, in both the scotopic (blue trace) and photopic (red trace) regions; see Eq. 20.1 below. However, the “dark light” that is described shortly is over 10,000× (4.1 log units) higher in the cone system than the rod system.

Scotopic vision: the rod system provides specialization for night vision
Light adaptation is examined under conditions that optimized detection by the scotopic (rather than photopic) system using the blue symbols of Fig. 20.1B . To achieve scotopic dominance, the test stimulus was presented in the peripheral retina, and comprised a large area, long duration green flash (9 degrees diameter, 200 ms, 520 nm) on a red background. The measurements are from Fig. 3 of Aguilar and Stiles and their troland values have been converted using a factor of K = 8.6 photoisomerizations s −1 per troland.
For comparison, the red symbols plot the desensitization of primate rod photoreceptors, obtained from Fig. 9A and Table III in Tamura et al. Their measurements of sensitivity have been converted to desensitization by taking the reciprocal; in addition, the symbols have been shifted vertically to provide a good fit to the psychophysical data in the high-intensity range.
The crucial difference between the blue and red symbols is that the overall scotopic visual system begins desensitizing at intensities around 1000 times lower than those required to begin desensitizing the rod photoreceptors. Thus, a considerable region at the lowest end of scotopic light adaptation is post receptoral, rather than being internal to the rods. Because the postreceptoral scotopic system is able to integrate photon signals from large numbers of rod photoreceptors, and thereby gain increased sensitivity, it needs to begin desensitizing at much lower background intensities to avoid saturation. As a result, the rod photoreceptors maintain their maximal sensitivity for several log units of the lowest-intensity regimen (up to approximately 10 isomerizations s −1 ) over which the visual system exhibits gradual desensitization.
When the background intensity is reduced from relatively high scotopic intensities (moving from right to left along the x-axis in Fig. 20.1B ) the desensitization of the rods, and of the scotopic visual system, steadily falls. However, below the intensities indicated by the red and blue arrows , the desensitization of first the rods and second the visual system fails to continue falling, as if the respective mechanism were experiencing a phenomenon equivalent to light. Accordingly, the arrowed intensities for the rods and for the scotopic visual system have been referred to as “equivalent background intensities,” or “dark light.” Clearly, the equivalent background for the scotopic system (around 0.016 photoisomerizations s −1 ) is more than 1000× lower than the equivalent background intensity for the rods (around 50 isomerizations s −1 ).
Each of the curves in Fig. 20.1 plots desensitization according to Weber’s law, although the curves in Fig. 20.1B additionally include saturation at high intensities, as described by the equation:
where Desens is desensitization, Desens D is its dark-adapted value, and I is the background intensity. The first term on the right expresses Weber’s law, where I 0 is the equivalent background intensity mentioned previously. This first term indicates that at low background intensities (when I << I 0 ) the desensitization approaches a constant level (its dark-adapted value, Desens D ), whereas for brighter backgrounds (when I >> I 0 ) the desensitization increases linearly with background intensity.
At higher scotopic intensities, both the rods and the overall scotopic system exhibit saturation, characterized by a steep rise in desensitization with increasing background intensity. This behavior is described by the second term on the right in Eq. 20.1 , where I sat is termed the saturation intensity. It is almost certain that saturation of the overall scotopic system results directly from saturation of the rods. Thus, for the blue and red curves in Fig. 20.1 B, the same value of saturation intensity, I sat = 2500 photoisomerizations s −1 (black arrow) , has been used. In contrast, the dark light is enormously different between the two curves, with I 0 = 0.016 and 50 photoisomerizations s −1 , respectively, for the psychophysical data ( blue arrow) and the rod photoreceptor data (red arrow) .
The span of intensities from I 0 (the dark light) to I sat (the saturation intensity) represents the Weber region, and in this range of background intensities the desensitization is directly proportional to background intensity. The reciprocal of desensitization is the sensitivity, S = 1/ Desens , and hence within the Weber region the sensitivity declines inversely with background intensity, i.e., S ∝ 1 / I .
Because the contrast in a visual stimulus is likewise inversely proportional to background intensity (i.e., contrast = Δ I / I ), this Weber region is characterized by a fixed level of contrast sensitivity, that is, a given level of contrast elicits a fixed size of response. Hence, an important feature of Weber law light adaptation is that it provides automatic extraction of visual contrast.
Fig. 20.1B shows that for the overall scotopic system the Weber region covers a very wide range, of at least 5 log units (i.e., over 100,000-fold).
Light adaptation of the electrical responses of cones and rods
In the presence of background illumination, it is not only the overall visual system that adapts—the rod and cone photoreceptors themselves display light adaptation, characterized by desensitization and acceleration of their electrical response to an incremental flash.
However, for rods, such adaptation occurs over only a restricted range of intensities before saturation sets in. Thus, the red data in Fig. 20.1B show that the Weber region for mammalian rods encompasses only 1 to 2 log units of intensity before saturation; for the larger rods of lower vertebrates the Weber region may encompass a slightly wider range of about 3 log units.
In contrast, cone photoreceptors undergo light adaptation over an enormously wide range of intensities, and they manage to escape saturation no matter how intense the steady light. In the overall photopic visual system it is likely that the observed adaptation results primarily from these changes at the level of the cones, rather than through postreceptoral processing.
Saturation of the electrical response in rods and its avoidance in cones
The response of a salamander rod to the onset of steady illumination at different intensities is illustrated in Fig. 20.2 . At the beginning of the step of light, the rod’s response begins rising according to the prediction from the time integral of the flash response (noisy traces). But under normal conditions (upper panel) the response very soon deviates, falling well below the linear prediction. Characteristically, the response to such a step of light typically exhibits an early peak followed by a sag? This deviation from the simplest linear prediction is a crucial aspect of light adaptation—if this deviation did not occur, then the rod would be driven into saturation by lights of very low intensity. Saturation can readily be induced by exposing the rod to a solution that clamps the cytoplasmic calcium concentration (lower panel) ; the measured responses then follow the predictions of the theoretical curves, and a very low intensity (labeled 2) saturates the rod. This result shows that at least a part of the rod’s ability to continue operating in backgrounds of moderate intensity is a consequence of changes in cytoplasmic calcium concentration; the molecular mechanisms that contribute to this will be discussed in section Molecular basis of photoreceptor light adaptation .

However, at higher background intensities, the circulating current in the normal rod becomes completely suppressed. Thus, for the upper panel of Fig. 20.2 , intensities higher than that labeled 4 (not shown) cause the response simply to rise to its maximum level, corresponding to the closure of all cGMP-gated channels in the outer segment, with the consequence that incremental stimuli are unable to elicit any incremental response, and the cell’s response is “saturated.” Typically, such saturation sets in exponentially with increasing background intensity, as described earlier by the second term on the right of Eq. 20.1
Cone photoreceptors exhibit even more pronounced “sag” from an initial peak when steady background illumination is turned on. An example is illustrated for modest steady intensities in the early region of the traces in Fig. 20.3 . The phenomenon is extremely robust in cones and, no matter how high the light intensity, the cone photoresponse always recovers to a reasonable operating level, even if it is transiently driven into saturation at the onset of intense illumination.

Desensitization and acceleration of the photoreceptor’s electrical response
In addition to showing the transient nature of the responses elicited by the onset of backgrounds in cones, the main purpose of Fig. 20.3 is to illustrate the desensitization of the responses to incremental flashes that is elicited by these backgrounds.
The traces illustrate the responses of a cone photoreceptor to an identical set of flashes presented under three different conditions. In Fig. 20.3A the flashes ( a – f , of progressively greater intensity) are presented in darkness. In Fig. 20.3B the same flashes are presented on a dim steady background, and in Fig. 20.3C they are presented on a brighter background. In the presence of background illumination, the responses to dim flashes are smaller. For example, for the second flash intensity, b , the response amplitude becomes markedly smaller from panel (A) to panel (B) to panel (C) of Fig. 20.3 . In other words, backgrounds of increasing intensity progressively desensitized the cone’s incremental response. Such behavior is very characteristic of photoreceptors (both cones and rods).
The changes in the dim-flash response elicited by backgrounds of different intensity are shown in Fig. 20.4A . The largest trace is the response to a dim flash presented under fully dark-adapted conditions, whereas the other traces are for the same flash presented on backgrounds of progressively brighter intensity. (In fact, to maintain responses of measurable amplitudes, the flash intensities were increased in the presence of backgrounds, and the traces actually plot response divided by flash intensity, i.e., response sensitivity.)

The traces in Fig. 20.4A demonstrate that the effect of increasing background intensity is both to desensitize and to accelerate the response to an incremental dim flash. This behavior of cones is very similar to that exhibited by rods, as illustrated in Fig. 20.4B .
Unaltered rising phase but accelerated recovery
For the incremental flash responses of the rod illustrated in Fig. 20.4B , the vertical scale has been adjusted to take account of changes in the level of circulating current remaining in the presence of the different background intensities. Thus, rather than plotting raw sensitivity (response per photoisomerization), as is done in Fig. 20.4A for the cone, Fig. 20.4B for the rod instead plots the fractional response (i.e., the incremental response as a fraction of the circulating current at that background) per photoisomerization. This has been done to provide a direct measure of the level of activation of the guanine nucleotide–binding protein (G-protein) cascade of phototransduction; thus, it can be shown that the level of cascade activation is best measured by the fractional channel opening, which in turn is measured by the incremental response expressed as a fraction of the existing circulating current.
When plotted in this manner, the incremental responses in Fig. 20.4B demonstrate the remarkable property that the onset phase of the response is invariant, (i.e., the traces for different background intensities exhibit a common rise at early times), indicated by the smooth black trace . This behavior indicates that the amplification parameter describing the activation steps in phototransduction is unaltered during light adaptation; in other words, light adaptation causes no change in the efficacy of the activation steps in phototransduction. Instead, what light adaptation does is to cause a marked speeding-up of the shut-off steps in the transduction cascade. The molecular identity of the steps that are accelerated is considered in section Molecular basis of photoreceptor light adaptation .
Dependence of sensitivity on background intensity: Weber’s law
By plotting the peak amplitude of each of the traces in Fig. 20.4A as a function of the background intensity on which it was measured, one obtains a sensitivity versus background plot of the kind illustrated in Fig. 20.5 .
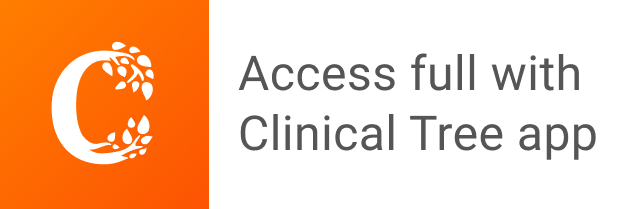