Overview
In the past decade, compelling evidence has emerged for a novel class of photoreceptors in the mammalian retina. These neurons are ganglion cells that express the photopigment melanopsin and respond autonomously to bright light with a sustained depolarization and increase in spike frequency. These intrinsically photosensitive retinal ganglion cells (ipRGCs) differ radically in form and function from the classical rod and cone photoreceptors. In this chapter, we discuss the origins of this discovery, the idiosyncratic physiology of these cells and roles they play in retinal processing and visual behavior in health and disease.
Historical roots
For most contemporary retinal scientists, the recent discovery of photoreceptors in the inner retina may have come as a shock, but the roots of the idea are actually traceable to the very beginnings of retinal science (see reviews ). Beginning with Descartes and through the first half of the 19th century, it was assumed that the photoreceptive elements of the retina lined its inner surface, nearest the incoming light. For example, Treviranus, the first to conduct systematic microscopic studies of the retina, suggested that optic fibers passed through all retinal layers to end as photosensitive papillae at the vitreal surface. Bidder accurately described the outer retinal location of the rods, but hypothesized that they served a reflective function, like a tapetum, and continued to view optic fibers in the inner retina as the locus of phototransduction. The lack of photosensitivity of the fibers of the optic disk, as indicated by the blind spot, eventually undermined this view, and prompted Bowman and Helmholtz to propose ganglion cells as the true photoreceptors. It was not until the latter half of the 19th century that photoreception was convincingly localized to the outer retina. The key insights were made by Heinrich Müller, who inferred the retinal depth of the photoreceptors by a clever geometric analysis of the parallactic displacement of shadows of retinal vasculature (the “Purkinje tree”). He also detected “visual purple” or rhodopsin in the rods, which Franz Boll soon thereafter demonstrated was a photosensitive pigment.
Fifty years would pass before interest in the possibility of inner-retinal photoreception would re-emerge. The catalyst was the groundbreaking work of Clyde Keeler, who identified the first animal model of inherited retinal degeneration. This strain of mice – which Keeler termed “ rodless ” – is allelic with the rd1 strain (formerly rd ); both carry the Pde6b rd1 mutation and phenotypically resemble human autosomal recessive retinitis pigmentosa (OMIM 180072). , Keeler eventually achieved wide acclaim for this seminal contribution to the genetics of retinal disease, but a key observation he made in these mice received little notice at the time. Though his rodless mice were apparently blind when tested behaviorally or electroretinographically, they unexpectedly retained robust pupillary responses to light. This led him to suggest that “in mammals the iris may function independent of vision” either through intrinsic photosensitivity of the smooth muscle itself or by “direct stimulation of the internal nuclear or ganglionic cells” by light. ,
Over the next 70 years, a series of studies in retinally degenerate mammals confirmed the persistence of the pupillary response and suggested that the functional blindness might not be as complete as Keeler had believed. Various photic effects on behavior or physiology were noted, despite the absence of an outer retina in histological material or a detectable electroretinogram (see reviews , ). These included avoidance of a visual cliff, photic suppression of activity in open field test, visually guided avoidance of shock in a shuttlebox, chromatic discrimination, entrainment of circadian rhythms, suppression of pineal melatonin synthesis, and suppression of spontaneous firing in the superior colliculus. A number of these studies demonstrated that eye removal abolished these residual photoresponses and overtly echoed Keeler’s speculation about the possible existence of inner retinal photoreceptors, including ganglion cells. These suggestions gained only limited currency, probably because improved anatomical studies had begun to cast doubt on the completeness of the loss of outer retinal photoreceptors, especially in the peripheral retina. These studies showed that the mouse retina, once believed to possess only rod photoreceptors, actually had a modest population of cones as well, and that these degenerated far more slowly than rods in the rd1 model. Perhaps 20 percent survived beyond 80 days of age, albeit without intact outer segments, and a few lasted at least a year. This clouded the interpretation of the behavioral studies, virtually all of which had been conducted in mice young enough to have had many surviving cones. With the benefit of hindsight, however, it seems plausible that at least some of the residual light-driven effects reported in these early studies were indeed mediated by inner retinal photoreceptors. Even in rd1 animals as young as 6–10 weeks old, surviving rods or cones seem unable to support visual function: silencing ganglion-cell phototransduction in such animals (as described below) abolishes the photic influences on circadian rhythms, the pupil, locomotor activity, and melatonin synthesis that these mice would otherwise exhibit.
In the 1990s, Russell Foster and colleagues took up the work on retinally degenerate mice, applying more rigorous methods to provide compelling evidence for inner retinal photoreception. Their innovations included appropriate controls for genetic background and the use of very old rd1 animals and several genetically modified mouse strains in which they confirmed nearly complete loss of cones as well as rods. They also showed that there was no loss of sensitivity of circadian photoreception as rod and cone degeneration progressed and that the residual visually evoked behaviors extended beyond circadian regulation to other outputs of what they called the non-image-forming (NIF) visual centers of the brain. These included pupillary constriction, acute suppression of locomotor activity and acute suppression of melatonin release by light. The case for novel inner photoreceptors was bolstered by parallel observations in human patients with advanced outer retinal disease and by assessment of the spectral tuning of residual photoresponses in retinally degenerate animals. Yoshimura & Ebihara showed that the photic influence on circadian rhythms in retinally degenerate mice was most effective at 480 nm, clearly distinct from the optimal wavelength for the known rod and cone photopigments. , Remarkably similar spectral data were obtained for residual pupillary responses.
Discovery of melanopsin and ganglion-cell photoreceptors
The identities of the mysterious inner-retinal photoreceptors and their photopigment were established in a flurry of studies in the years 2000 to 2005. These developments have been thoroughly reviewed elsewhere, so we provide only an abbreviated summary here. A pivotal contribution was the discovery of melanopsin. , This novel opsin (coded by the opn4 gene) derives its name from the dermal melanophores of frogs, the photosensitive cells of the skin in which the gene was first identified. Ignacio Provencio and colleagues showed that in mice and primates, including humans, the protein was expressed solely in a small minority of cells in the inner retina, mainly in the ganglion cell layer. They speculated that melanopsin could be the photopigment of the postulated inner-retinal photoreceptors and that the neurons expressing it might be the cells of origin of the retinohypothalamic tract. These ideas were at odds with an alternative suggestion, introduced a few years earlier, that the relevant photopigment might not be an opsin but, rather, a cryptochrome, a blue-light-absorbing flavoprotein (see reviews , ). However, overwhelming evidence for melanopsin’s central role soon emerged from studies in rodents and in heterologous expression systems.
Two key studies in this cohort focused on rat retinal ganglion cells shown by retrograde axonal tracing to innervate the suprachiasmatic nucleus (SCN) of the hypothalamus, the brain’s circadian pacemaker. The first showed that these ganglion cells expressed melanopsin, while the second demonstrated that they generated robust electrical responses to light even when pharmacologically or mechanically isolated from other retinal neurons. , Because this capacity for autonomous phototransduction distinguishes these cells from all other RGCs, they are termed intrinsically photosensitive retinal ganglion cells (ipRGCs) or ganglion-cell photoreceptors. Both mice and primates were also soon shown to possess ipRGCs with structural and functional properties much like those in the rat (see Box 26.1 ).
Ocular enucleation and evisceration are common surgical therapies for managing chronic debilitating ocular pain and improving cosmesis of blind eyes. , Such surgery is typically indicated only in eyes lacking useful vision. However, the recent discovery of inner retinal photoreceptors should prompt a reassessment of best practices in such cases. When the blindness is attributable to outer retinal diseases such as retinitis pigmentosa or Leber’s congenital amaurosis, or to chronic retinal detachment, ganglion-cell photoreceptors may continue to provide functionally useful signals to the brain. These signals may not be consciously perceived by the patient and may escape detection by most standard clinical tests of visual function. Such residual functions can nonetheless have significant impact on the quality of life for blind patients, since they may entrain their circadian rhythms. Loss of ipRGCs through enucleation or evisceration would be expected to abolish such synchronization of circadian rhythms, with associated disruption of normal sleep patterns, daytime sleepiness, and other complications that plague many blind patients. It seems likely that photic effects on physiology, including regulation of neuroendocrine physiology, alertness and mood, may also persist in such patients and be sacrificed when the eyes are removed. There is thus a pressing need to develop both a more inclusive concept of “useful vision” in ocularly blind patients and a more comprehensive panel of assessments of photosensory capacity when enucleation or evisceration is being weighed as a treatment option. Although tests of circadian or neuroendocrine effects would in principle allow residual inner retinal photoreceptor function to be evaluated, these would require highly specialized testing. Assessments of residual pupillary responses may be a promising avenue to explore.
The presence of melanopsin within physiologically identified ipRGCs was first demonstrated directly by Hattar et al . The opsin was found not only in the cell body, but also in their dendrites , which, like the soma, are directly photosensitive. Further support for the view that melanopsin was the sensory photopigment in these cells came from the opsin-like action spectrum of the light response in ipRGCs and from the observation that the intrinsic photosensitivity of these cells was abolished in melanopsin knock-out mice. , The capacity of melanopsin to function as a sensory photopigment was first demonstrated biochemically, and subsequently confirmed by electrophysiology and calcium imaging in heterologous expression systems. There is remarkably good concordance in the spectral sensitivity in this system, as assessed from absorbance of heterologously expressed or purified melanopsin, from the action spectrum of ipRGCs, and from behaviors mediated by inner retinal photoreceptors; all closely adhere to a retinaldehyde template function with a best wavelength near 480 nm 2 (but see refs & ).
Distinctive functional properties of ipRGCs
Ganglion-cell photoreceptors have physiological properties that are optimized for their roles in NIF vision and contrast markedly with those of the rod and cone photoreceptors that feed the cortical circuits mediating the perception of form, color and motion.
Melanopsin chromophore and pigment bistability
In both vertebrate and invertebrate photopigments, the opsin apoprotein is covalently linked to a retinaldehyde molecule derived from vitamin A that serves as the light-absorbing moiety, or chromophore. In darkness, the retinaldehyde is in the 11- cis form. Absorption of a photon converts it into all- trans retinaldehyde. This triggers a conformational change in the opsin which in turn activates a G-protein, initiating the transduction cascade. Before the photopigment can undergo another cycle of photoexcitation, the all- trans retinaldehyde must be reisomerized to 11- cis . For rod and cone photoreceptors, such reisomerization is carried out through multiple enzymatic steps. Several of these occur in cells of the retinal pigment epithelium (RPE), which are essential for the visual cycle in rods and important, if not obligatory, for cones (see Chapter 13 for more information). The visual cycle in invertebrate photoreceptors appears to be radically different, in part because their photopigments are bistable: after photoexcitation, all- trans retinaldehyde remains covalently bound to the opsin and is reisomerized to 11- cis by subsequent absorption of light in a process known as photoreversal.
The visual cycle of melanopsin in ipRGCs has not been well characterized. The chromophore of melanopsin in situ is unknown, but is doubtless a retinoid, and is most likely to be 11- cis retinaldehyde. Sequence homology indicates that melanopsin resembles invertebrate opsins more than it does vertebrate ones, , implying that it might be bistable. There is growing evidence in support of this view (but see ref. ) . If melanopsin is indeed bistable, it would be expected to be less reliant than rods or cones on the enzymatic machinery of the RPE for the regeneration of its chromophore, and might be utterly independent. Indeed, ipRGC photoresponses can be recorded for many hours in vitro in retinas isolated from the RPE. Furthermore, neither acute pharmacological disruption of the retinoid cycle nor genetic deletion of the essential isomerohydrolase of the RPE (RPE65) abolishes the melanopsin-based photoresponse of ipRGCs or their ability to photoentrain circadian rhythms , (but see ref. ). The evidence implies that to a remarkable degree, the melanopsin in ipRGCs can be loaded with its chromophore and can recover from photoactivation independent of retinoid processing by RPE. Melanopsin might acquire its retinoid mainly in the form of all- trans retinal which it then photoisomerizes to a cis isomer to form a photoexcitable pigment. This autonomy is important for the intrinsic photosensitivity of ipRGCs, because these cells are located far from the RPE (see Box 26.2 ).
There are at least two clinical conditions in which disruption of normal interactions between RPE and neural retina compromise rod and cone function but may permit continued phototransduction by ipRGCs. Retinal detachment disrupts the close contact between rod and cone outer segments and the RPE. This compromises the bidirectional exchange of retinoids across the subretinal space and thus the photosensitivity of rods and cones. Leber’s congenital amaurosis (LCA), an autosomal recessive, early-onset form of retinitis pigmentosa, is caused in some cases by mutations in the rpe65 gene. This disrupts the function of RPE65, a retinoid isomerase essential for the regeneration of 11- cis chromophore for rod and cone photopigments. Both retinal detachment and LCA have devastating consequences for photosensitivity of rods and cones. Evidence from animal studies (see main text) predicts that photosensitivity of ipRGCs should be substantially preserved under these conditions and should thus provide some useful photic information to the brain.
Spectral tuning
As noted above, melanopsin has peak sensitivity in the blue region at around 480 nm, different from the spectral sensitivities of the rod and cone visual pigments ( Fig. 26.1 ). This wavelength roughly coincides with the peak of solar emission, perhaps the result of evolutionary pressures to optimize the sensitivity of this system to daylight. Though melanopsin is often described as a blue-light-sensitive pigment, it is important to recognize that, like other opsin-based photopigments, its spectral tuning is rather broad. Throughout the spectral range from 340 to 590 nm, sensitivity is within 2 log units of that seen with the optimal wavelength ( Fig. 26.1 ). Though there is evidence that ipRGCs project to the lateral geniculate nucleus (LGN) and could thus contribute to cortical function, , we are unaware of any evidence that the unique spectral tuning of melanopsin is exploited in the conscious appreciation of color, which is well accounted for by the three cone photopigments and trichromatic theory (see Box 26.3 ).

Designers of intraocular lens implants must grapple with the fact that there are both harmful and salutary effects of short-wavelength visible light. The goal must be to strike the appropriate balance between photoprotection and photoreception. High-pass filters that block blue as well as ultraviolet wavelengths offer better retinal photoprotection than those that block only ultraviolet light, while leaving rod and cone photoresponses (which peak near 500 nm and 555 nm respectively) relatively unaffected. However, because melanopsin is most sensitive to blue light with peak sensitivity at 480 nm, lenses that block blue wavelengths can compromise NIF photoreception. On the other hand, reducing NIF photoreception in this manner might have the benefit of reducing the harmful effects of light exposure at night on the NIF visual system, as discussed in the main text below. These factors need to be considered in the design and selection of lens implants. A recent study showed that for the typical person, long-pass filtering with a sharp filter cutoff near 445 nm may provide the best compromise between photoprotection and light reception.
The spectral behavior of the ipRGC output signal is much more complex than predicted simply from the action spectrum of the intrinsic light response as measured in dark-adapted ipRGCs. This is in part because melanopsin appears to be a bistable photopigment (see the previous section). The absorption spectrum of the activated form of the pigment, and thus of photoreversal, is shifted to longer wavelengths with respect to that of melanopsin in the dark state (e.g. refs & ). How this shapes the ipRGC output signal under physiologically relevant lighting conditions is still in dispute, but it suggests the possibility of a form of spectral opponency under some conditions. A second source of complexity and potential spectral opponency is the functional input to the ipRGCs from classical rod and cone photoreceptors, discussed in detail below. Because the threshold for melanopsin activation is above that for rods and cones, the spectral tuning of ipRGCs can be expected to be intensity dependent. Furthermore, there is evidence in primates that the cone input to ipRGCs is itself spectrally opponent, with activation of short-wavelength cones driving OFF responses in ipRGCs, and activation of mid- and long-wavelength cones driving ON responses.
Invertebrate-like phototransduction cascade
In a photoreceptor cell, the light-absorbing pigment signals through an intracellular biochemical pathway to transduce light into electrical activity across the cell membrane. Interestingly, in ipRGCs this phototransduction cascade is more similar to those in invertebrate photoreceptors than to those in rods and cones. We have already noted that melanopsin has greater sequence homology with invertebrate photopigments than with the rod and cone opsins. , Upon stimulation by light, melanopsin activates a G-protein belonging to the G q/11 family, which in turn signals to the effector enzyme phospholipase C (PLC). PLC then somehow causes the opening of a cation channel, which has been proposed to belong to the family of transient receptor potential (TRP) channels. While the signaling intermediates coupling PLC to the cation channel remain to be elucidated, the critical components are likely to be within or closely associated with the plasma membrane because patches of cell membrane isolated from ipRGCs continue to exhibit light-evoked electrical responses. Thus, the entire ipRGC phototransduction cascade is very similar to that in Drosophila photoreceptors but differs drastically from the rod/cone cascade (see also Chapter 18 ) ( Fig. 26.2 ). This invertebrate-like phototransduction cascade has prompted speculation that ipRGCs and invertebrate photoreceptors share a common evolutionary origin. It also confers upon ipRGCs properties well suited to NIF vision, as discussed below.

Depolarizing photoresponse with action potentials
The endpoint of the melanopsin phototransduction cascade is the opening of ion channels in the plasma membrane. These behave like cation-selective channels. , The interior of an ipRGC is negatively charged relative to the extracellular space so the opening of light-gated channels results in net influx of cations and membrane depolarization. In this ipRGCs once again resemble invertebrate rhabdomeric receptors, which likewise depolarize when illuminated, and differ from the vertebrate rods and cones, which are hyperpolarized by light. If the depolarizing ipRGC light response is large enough, it brings the membrane potential above the threshold for activating voltage-gated sodium channels, triggering action potentials ( Fig. 26.3 ). This represents yet another divergence from the rod/cone photoreceptors, which do not spike. Action potentials are necessary for ipRGCs, as for other RGCs, to propagate electrical information faithfully along the optic nerve and tract to relatively distant central visual centers. By contrast, rod and cone axons are very short, and passive spread of the electrical signals generated in the outer segment is sufficient for appropriate voltage modulation of the axon terminal.

Sensitivity
A salient feature of ipRGCs is the relative insensitivity of their melanopsin-mediated responses to light. The threshold intensity for the ipRGC intrinsic photoresponse is 1 to 2 orders of magnitude (i.e. 10–100 times) higher than that for cones, and up to 5 log units higher than that for rods. Such insensitivity is apparently due mainly to the low abundance of melanopsin molecules in ipRGCs, and the resulting low probability of photon absorption. This in turn is related to the fact that ipRGCs lack any structural specialization for increasing the packing of pigment-laden membrane in the cell, such as the disks in the outer segments of rods and cones or the rhabdomeric microvilli of invertebrate photoreceptors. The insensitivity of intrinsic, melanopsin-based responses in ipRGCs means that such responses are triggered mainly by bright sunlight during the day, which is the most effective stimulus for circadian photoentrainment and full pupillary constriction. Because the dynamic range of these responses complements those of the rod and cone photoreceptors, and because all three of these signals converge on the ipRGCs (see below), the ipRGCs and at least some of the NIF visual responses they drive are able to function over the entire intensity range of naturally encountered light stimuli. ,
Kinetics
The temporal characteristics of the melanopsin-based ipRGC photoresponse are drastically different from those of the rod and cone photoreceptors, with remarkably slower onset and termination, and great stability in the face of sustained illumination. Rod and cone photoresponses begin within several milliseconds of light onset and peak within tens of milliseconds. Even the fastest ipRGC intrinsic responses, evoked by very high light intensities (>10 14 photons/cm 2 /sec at 480 nm), are much slower, with the first action potential appearing no earlier than a few hundreds of milliseconds after light onset and peak firing rate achieved only after several seconds. Latencies rise dramatically for less intense flashes, and for near-threshold light stimuli (~10 11 photons/cm 2 /sec at 480 nm) can be as long as tens of seconds to the first spike and several minutes before the peak discharge is reached. The basis for such sluggish onset is unknown, but it is of interest that when melanopsin was heterologously introduced into three different cell culture systems, the photoresponses recorded had very slow onset in all three cases. Such sluggishness appears unlikely to be an intrinsic property of melanopsin, because single-photon responses in ipRGCs reportedly have relatively brisk onsets. The long latencies, especially near threshold, seem more likely to result from the temporal integration of many low-amplitude, long-lasting, single photon events.
In response to a prolonged light flash, the melanopsin-driven response gradually decays from an early peak to a lower steady-state level. This reflects light adaptation, which prevents saturation of the phototransduction cascade. After light adaptation is complete (which typically requires several minutes), membrane potential and thus spike frequency remain elevated for as long as the light stimulus persists. Analysis of activity-dependent induction of the immediate early gene cfos in putative ipRGCs implies that these photoreceptors can respond continuously to light for at least 19 hours. Thus, the ipRGC intrinsic photoresponse is far more sustained ( Fig. 26.3 ) than the cone response, which adapts much faster and more completely, making the response very transient. The ability of ipRGCs to continuously signal the presence of bright light for many hours is a key feature of the NIF visual system, which features tonic responses to steady diffuse illumination and the ability to integrate photon flux over periods of more than an hour, as explained in further detail below.
The termination of the melanopsin light response is extraordinarily slow. After the cessation of light stimulation, cone and rod responses terminate within hundreds of milliseconds and several seconds, respectively. But termination of the intrinsic photoresponse of ipRGCs takes up to several minutes, especially for very high light intensities. The reason for this slow recovery is not established, but the similarity of melanopsin to invertebrate rhabdomeric opsins suggests that it results in part from the thermal stability of the light-activated (metarhodopsin) form of the photopigment. , If so, the slowly decaying post-stimulus voltage response is directly analogous to the persistent depolarizing afterpotential of invertebrate photoreceptors. This is supported by the observation that the poststimulus depolarization can be suppressed by exposure to long-wavelength light, presumably by triggering photoreversal of the pigment. However, light is not required for response termination, because this can occur in complete darkness. The light-independent mechanisms for response termination are unknown. By analogy to other better characterized phototransduction cascades, however, they seem likely to involve phosphorylation of melanopsin followed by arrestin binding.
Morphology, retinal distribution and receptive field
In all mammals studied to date, melanopsin-expressing retinal ganglion cells represent a small minority of all RGCs. These are now recognized as comprising multiple cell types. The best studied type has a very large, sparse dendritic arbor stratifying in outermost inner plexiform layer (IPL), abutting the inner nuclear layer (INL). In mouse and primate retinas, there is an additional wide-field melanopsin ganglion cell type stratifying in inner IPL ( Fig. 26.4 ). In humans, there are roughly equal numbers of these two cell types, and most of the outer-stratifying type have somas displaced to the INL. Bistratified cells have also been described, although it is not clear if these define a distinct cell type. Recent evidence in the mouse suggests there are several additional types with very low levels of melanopsin expression. Differences among these cell types in functional properties or projections to the brain are just beginning to be explored. , A very few human cones of the far retinal periphery have been reported to express melanopsin, but their functional significance is unknown.

The morphology and distribution of human ipRGCs have been described in detail. , Of the ~1.2 million RGCs in humans, only about 3000 (about 0.2 percent) express melanopsin. The somas of these cells are relatively large in primates, typically 15–25 µm in diameter. Their dendritic fields are the largest of any ganglion-cell type, ranging from 300 µm in the central retina to 1200 µm in the periphery ( Fig. 26.4 ). The dendrites of neighboring cells overlap extensively so that the entire retina, except for the fovea, is covered by a network of melanopsin-containing processes ( Fig. 26.5 ). Because both the cell bodies and the dendrites of these cells are intrinsically photosensitive, , each ipRGC integrates photons over a region comparable to its dendritic field size. These large receptive fields and low spatial densities make ipRGCs ill-suited for fine spatial discriminations, but ideal for the pronounced spatial integration that characterizes NIF functions. They contrast sharply with the very small receptive fields of rods and cones (<10 µm in diameter), the foundation for the fine-grained retinal representations at the cortical level that permit high acuity spatial vision.

Resistance to pathological states
Several studies have suggested that ipRGCs are more resistant to certain types of retinal damage than are other ganglion-cell types. For instance, after axotomy, melanopsin-expressing RGCs have a higher survival rate. In addition, melanopsin RGCs are less susceptible to artificially induced chronic ocular hypertension in Sprague-Dawley rats, although their number are certainly reduced. Furthermore, ganglion cells exhibiting cFos induction by light (presumably including ipRGCs) were much less vulnerable to systemically administered monosodium glutamate, suggesting that ipRGCs are relatively resistant to excitotoxicity. The molecular basis for these kinds of resistance is largely unknown, although the PI3 K/Akt signaling pathway appears to be involved. Further investigation into the molecular basis of ipRGC survival after retinal injuries may lead to novel strategies for preventing and/or treating various retinal diseases (see also Box 26.4 ).
Transient transfection with the melanopsin gene is sufficient to induce photosensitivity in a variety of cell types in culture ( Fig. 26.6 top ). Introduction of melanopsin into inner retinal cells thus holds promise as a candidate gene therapy for restoring sight in retinitis pigmentosa and other forms of outer retinal blindness. The practicality of this approach is enhanced by the fact that ipRGCs do not depend upon the RPE for chromophore regeneration. Another advantage is that all of the critical elements of melanopsin-driven phototransduction appear to be associated with the plasma membrane, thus reducing the requirements for specialized cytosolic machinery. Indeed, two recent studies using a mouse model of retinitis pigmentosa report that transfecting conventional RGCs with the melanopsin gene renders most of them photosensitive and can rescue some light-driven behavioral responses , ( Fig. 26.6 bottom ).

However, several disadvantages of this approach need to be considered. First, in both ipRGCs and the various heterologous expression systems, the melanopsin-based photoresponse is far more sluggish than those of the rods and cones. Thus, even if image-forming vision is restored, the patient’s perception of stimulus motion and of the timing of transient visual events would presumably be highly distorted. Second, ipRGCs are even less sensitive to light than cones, so visual threshold would be even higher than for people suffering from night blindness. Still, melanopsin is ~3–5 log units more sensitive than the most promising alternative candidate for gene therapeutic induction of inner-retinal photosensitivity, namely the microbial light-gated ionophore channelrhodopsin-2. , Threshold intensities are approximately 10 11 photons/cm 2 /sec for melanopsin as compared to ~10 14 to 10 16 photons/cm 2 /sec for channelrhodopsin-2. Third, if melanopsin is targeted to RGCs, all of which have dendritic fields vastly larger than the profile of rod and cone outer segments, then spatial resolution would be quite poor. The average receptive field diameter of melanopsin-transfected mouse RGCs in one study was about 100 µm. To improve spatial resolution, it might be better to selectively target bipolar cells for melanopsin transfection, though it is unknown if these cells express critical components of the melanopsin phototransduction cascade. Even if they do, sensitivity is likely to be an even greater concern than in ganglion cells; the relatively small area of surface membrane in bipolar cells would presumably limit the number of melanopsin molecules available for photon absorption.
The PLR has potential utility as a diagnostic tool to differentiate between outer retinal disorders affecting rod or cone circuits and inner retinal diseases affecting ganglion cells. As illustrated in Fig. 26.12 , components of the PLR mediated by the rod and cone photoreceptors are temporally separable from those mediated by melanopsin. The earliest component of the light-driven constriction is mediated almost exclusively by rods and/or cones. By contrast, the slowly decaying post-stimulus constriction is driven by melanopsin; this presumably reflects the persistent depolarizing after-potential in ipRGCs that, in turn, is thought to be supported by a slowly depleting pool of photoactivated (meta) melanopsin (see “ Melanopsin chromophore and pigment bistability ” in the main text above). It should also be possible to tease out specific photoreceptor contributions by exploiting differences among them in threshold and saturating intensities and in spectral sensitivity. Red light may be presented under rod-saturating photopic conditions to selectively evoke a cone-driven PLR, whereas bright blue light is most effective for inducing a melanopsin-mediated response that persists beyond stimulus termination. To elicit a response driven mainly by the rods, a dim green light presented under a dark-adapted condition is ideal.
Synaptic input
Unlike conventional RGCs, ipRGCs do not require synaptic input to respond to light because they are directly photosensitive. Nonetheless, both primate and rodent ipRGCs receive synaptic inputs from amacrine and bipolar cells, and these have consequences for the timing, spectral behavior and sensitivity of the light-evoked discharges of these cells. A schematic diagram summarizing the intraretinal synaptic inputs to ipRGCs appears in Figure 26.7 .

Bipolar cell input
There is strong structural and physiological evidence for synaptic contacts from bipolar cells onto ipRGC dendrites. The earliest anatomical evidence came from electron-microscopic immunohistochemical data in mice showing ribbon synaptic (bipolar) contacts onto melanopsin-expressing dendrites and this has been supported by subsequent structural observations. Bipolar-cell input to ipRGCs has also been confirmed electrophysiologically. Rodent ipRGCs are excited by applied glutamate, the transmitter released by bipolar-cell terminals. Spontaneous glutamate-mediated excitatory postsynaptic currents are detectable in ipRGCs, and these almost certainly derive from bipolar cell synapses. , Under appropriate recording conditions, white light elicits two excitatory ON response components in ipRGCs. The first has a high threshold, is sluggish in its onset and termination, and is insensitive to synaptic blockers; these properties testify to its origin in intrinsic melanopsin-based phototransduction. The other component occurs much more rapidly after light onset, can be abolished by pharmacological blockade of synaptic transmission, and is attributable to excitatory synaptic input from ON bipolar cells. Both rods and cones contribute to these extrinsic light responses, making them about five orders of magnitude more sensitive to light than the melanopsin photoresponse , ( Fig. 26.8 ).
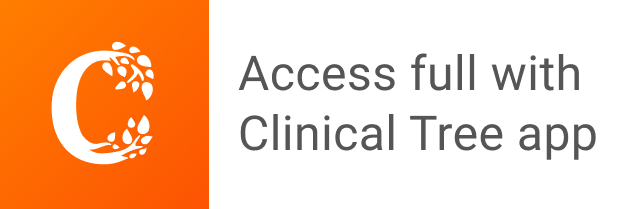