What is extrastriate visual cortex?
The term “extrastriate” refers to all visually responsive cortex other than primary visual (striate) cortex, and does not receive strong direct projections from the lateral geniculate nucleus (LGN) (see Chapter 30 ). A central hypothesis in studying extrastriate cortex is that it is composed of discrete cortical areas which can be identified by histology, retinotopic mapping, patterns of connections with other areas, and the unique contribution each area makes to visual processing. Extrastriate areas that receive strong inputs directly from primary visual cortex can be thought of as a lower tier within a processing hierarchy, compared to a higher tier of areas that receive their main visual inputs from relays through other extrastriate areas. The more relays between an extrastriate area and primary visual cortex, the higher its position in the processing hierarchy and, generally speaking, the more complex is its visual processing. Superimposed on this hierarchy is the concept of parallel streams of visual areas that are more strongly interconnected with each other than with areas of different streams, and that are all concerned with some common aspect of visual processing, such as object recognition (ventral stream) or object location (dorsal stream). At least 25 cortical areas predominately or exclusively engaged in vision have been described in monkeys; the number of visual areas identified in humans is rapidly approaching, and will probably surpass, that total ( Fig. 31.1 , Box 31.1 and see also Box 31.6 ). ,

“Lower tier” extrastriate visual areas receive predominantly from primary visual cortex.
“Higher tier” extrastriate areas receive less from primary visual cortex, and more from both lower and higher tier areas and generally have more complex visual processing.
Lower Tier Areas
- •
V2
- •
V3
- •
MT
- •
PO
Higher Tier Ventral Stream Areas (Object Recognition)
- •
V4
- •
PIT
- •
CIT
- •
AIT
- •
TH
- •
TF
Higher Tier Dorsal Stream Areas (Motion and Location of Objects)
- •
MST
- •
LIP
- •
7a
- •
VIP
- •
AIP
- •
Extrastriate visual cortex contains a constellation of richly interconnected areas comprising large portions of the occipital, parietal, and temporal lobes and contributes to a diverse array of visual information-processing tasks
- •
Extrastriate visual areas are identified by a combination of features including receptive field mapping, histology, patterns of connections with other brain regions, and functional specificity
- •
In both monkeys and humans a dorsal visual pathway dealing with object location extends through V2, V3, MT, PO/V6, and into parietal cortex
- •
In both monkeys and humans a ventral pathway dealing with object recognition extends through V2, V4, and into temporal cortex
Methods used to identify extrastriate areas in monkeys and humans
Identifying cortical areas and their borders is neither straightforward nor completely resolved, and different schemata for parceling extrastriate cortex into areas exist, depending upon how the criteria of histology, retinotopy, connectivity, and physiology are weighted. There is most consensus regarding areas lower in the hierarchy, nearer to V1, and most divergence of opinion for higher-level areas in the temporal and parietal lobes.
Histology
Cortical areas can be expected to have a uniform and distinct histology, such that anatomical borders can be recognized by changes in the laminar organization, density, or size of neurons, myelinated fibers, enzyme activity, neurotransmitter levels and their receptors, and so forth. However, not all borders can be recognized with all stains. Early parcellation schemes based on classical histological techniques are still in wide use, although containing many known inaccuracies. Based on Nissl staining of neuronal cell bodies (cytoarchitectonics), Brodmann divided most of extrastriate occipital cortex, in both human and monkeys, into only two areas, 18 and 19, each of which includes multiple areas defined by other criteria such as retinotopy and connectivity. Other histologic stains not available to Brodmann, such as cytochrome oxidase (CO; a metabolic enzyme that serves as a marker for long-term activity levels of neurons) histochemistry, reveal the borders of the many visual areas within the cortical territory occupied by Brodmann’s areas 18 and 19. Even with improved staining techniques, however, it is likely that some areal boundaries may escape detection by any histologic methods.
Retinotopic mapping
Each visual area comprises a single map of receptive field locations, with V1 demonstrating the most complete map of visual space (see Chapter 30 ). These maps can be studied with electrophysiologic recordings in monkeys and with functional magnetic resonance imaging (fMRI) or positron emission tomography (PET) in humans. However, none of the extrastriate maps are as regular as in striate cortex (V1). Extrastriate areas have larger receptive fields and more scatter in the position of each receptive field, resulting in “cruder” maps. Between extrastriate areas, there is generally a smooth transition of receptive field locations. Contiguous visual areas can have “mirror-image” representations, where the topography of the visual field map is reversed at the border of the two contiguous areas. When a mirror-image area like V1 borders a non-mirror-image representation like V2, the vertical meridian representation forms the border of the two areas and allows continuity of receptive field locations across the border. Visual field sign (mirror image or non-mirror image) can be computed from the local gradient of receptive field position, and can be used to infer borders of extrastriate areas. Extrastriate maps also tend to show breaks in the retinotopy (split representations) along the horizontal meridian representation ( Fig. 31.2 ). These breaks often form the borders of visual areas, allowing smooth transitions of receptive field locations from one area to the next, at the expense, in this case, of splitting the receptive field map within a single area. The splitting of maps within a single area and the continuity of receptive field positions at the borders of different areas make it difficult to identify areal boundaries on the basis of receptive field maps alone.

Connection patterns
Each visual area is interconnected with a subset of the other visual areas and subcortical visual structures, giving it a unique connectional signature. Neuroanatomic tracing methods in monkeys involve injecting tracers into one area either to label cells projecting to the injected area or to label axons projecting from the injected area to other areas. Tracing connectivity in human visual cortex was classically limited to postmortem staining for degenerating axons after cortical lesions. Two new techniques suitable for studying connectivity in human extrastriate areas have recently been developed. Connections can be traced between cortical areas with an MRI technique that analyses the anisotropy of diffusion of water within oriented bundles of axons (diffusion tensor analysis; DTI). , Currently, DTI is not, and may never be, sensitive enough to demonstrate all connections among visual areas, , but it has proved useful in demonstrating pathological changes. , Connectivity can also be inferred by imaging a rise in activity of target areas following noninvasive activation of a projecting cortical area with transcranial magnetic stimulation (TCMS). TCMS induces a brief electrical current inside cortical neurons by external application of a large, rapidly fluctuating magnetic field, and, with proper stimulation paradigms, can either activate or inactivate cortical areas. It is important to note that connections are between retinotopically corresponding points in the visual field representation of the interconnected areas. Thus connectivity information has been used to infer receptive field maps. This is especially true for interhemispheric connections, which, especially for areas in the lower tier of the processing hierarchy, are concentrated in representations of the vertical meridian.
Functional specificity
The functional properties of neurons are often characteristic of each extrastriate area. A range of visual stimuli, selected to emphasize, for example, motion versus form and color information, can be used to selectively activate specific areas. These studies, like those detailed above on retinotopy, can be performed in humans using functional imaging techniques (fMRI, PET) or in monkeys using imaging or electrophysiological techniques. In vivo imaging in humans can reveal functional deficits due to cortical lesions caused by stroke, and localize the site of the damage to particular cortical areas. Repetitive TCMS can be used to reversibly inactivate extrastriate cortical areas in humans, allowing deficits in function to be associated with the inactivated area. ,
Comparing visual areas in monkeys and humans
Related to the problem of identifying different areas in a single species is that of identifying homologous visual areas in different species. The functional organization of cortical areas has been studied extensively in monkeys, but it has been only with the recent advent of new non-invasive methods, that comparable studies have been done in humans. Most non-human data are from a genus of Old World monkeys ( Macaca sp.). Although these species are more closely related to apes (including humans) than other primate groups (e.g. New World monkeys and prosimians), at least 25 million years of evolutionary history separate humans and macaque monkeys, with many new cortical areas being added in humans. Lower-level areas (V1, V2, V3d/VP, and MT) appear to be present in all primates, including humans, but there are important species differences in cortical organization for higher-level visual areas. In addition to similarities in histology, retinotopy, connectivity, and physiology, relative position on the cortical surface and with respect to common sulcal and gyral landmarks is used to compare human visual areas with those of monkeys. This is not straightforward, however, as borders of cortical areas relative to sulcal landmarks vary among individuals, even more so in humans than in monkeys. Moreover, there is no clear human analog of the macaque monkey’s lunate sulcus, in which lie visual areas V2, V3, and V4. Comparisons between human and monkey extrastriate cortex are also made more difficult by the fact that human V1 does not extend onto the external aspect of the hemisphere as much as in monkeys; accordingly, all of the human extrastriate areas appear displaced posteriorly relative to their positions in monkeys. The best positional clues to correspondence between monkey and human extrastriate areas are from each area’s location relative to other cortical areas, which does seem to be relatively well conserved.
Processing streams in extrastriate cortex
As nearly all of the projections from the LGN terminate solely in striate cortex, the extrastriate areas receive their visual inputs through area 17, either directly or indirectly. (Some extrastriate areas do receive minor thalamic inputs from the lateral geniculate body , and the pulvinar, which may contribute to blindsight. ) The main extrastriate projections of V1 are to V2, V3, V4, and MT. As mentioned earlier (see Chapter 30 ), these projections arise from different subsets of neurons in V1 with different laminar and columnar distributions and likely different functional properties. Zeki first pointed out that striate cortex thus acts to initially segregate and then recombine the visual inputs of the three retinogeniculocortical pathways, magnocellular, parvocellular, and koniocellular, such that different visual information is parceled out to different extrastriate cortical areas for further analysis. As described in Chapter 30 and shown in Figure 31.3 , projections from V1 give rise to two main streams of extrastriate cortical processing, a dorsal stream devoted to spatial vision (“where” stream) and a ventral stream devoted to object vision (“what” stream). As in V1, there is often a tangential organization of the inputs and outputs from different streams, forming a mosaic pattern when viewed from the cortical surface. This columnar and tangential organization of connectivity is often marked by differences in histochemical, particularly CO, staining.

V2
In nearly all mammals, the second visual area, V2, borders V1 laterally and dorsally, extending around V1 nearly completely in the case of primates. V2 has a mirror-image visual field map, with the representation of the vertical meridian forming the boundary with V1 and a split representation of the horizontal meridian forming the boundary with V3d dorsally and VP ventrally. V2 functions closely with V1 to organize information for output to higher visual areas such as V4 and MT ( Box 31.2 ).
- •
Part of both the “what” and “where” streams
- •
Composed of thick, thin, and pale CO stripe compartments
- •
Thin stripes receive from CO blobs of V1 and project to V4; unoriented and color-selective receptive fields predominate
- •
Thick stripes receive from the interblobs of V1 and project to MT; orientation and retinal disparity-sensitive receptive fields predominate
- •
Pale stripes receive from the interblob regions of V1 and project to V4; orientation-selective receptive fields predominate
- •
Local connections in V2 interconnect all CO stripe compartments
- •
In humans, V2 is split into four quadrants, with an upper and lower visual field representation in each of the two hemispheres
- •
Lesions in V2 give rise to selective visual loss depending on the CO stripe compartment affected
The segregation of inputs, outputs, and physiological properties within area V2 is as dramatic as within V1 and, as in V1, is associated with a special pattern of CO staining. CO staining in sections of monkey V2 shows a series of parallel stripes of alternating dark and light staining that are oriented perpendicularly to the V1/V2 border. , , The V2 stripes, which are larger than the blobs in V1, extend across the whole width of the area, allowing its borders to be demarcated histologically using the CO stain. The darkly staining stripes differ in thickness, with thin and thick stripes alternating, thus defining three compartments (thick, thin, and the pale interstripes) within V2. Sections through human V2 also reveal a striped organization when stained for cytochrome oxidase and other histological stains. The stripes are not as regular as in monkeys, and differentiation into thick and thin stripes is not clear based on CO staining. However, histologic stains known to differentiate thick and thin stripes in monkeys demonstrate a clearer stripe organization , with a periodicity of about 0.7–1 cm for a complete set of stripes, several times larger than in monkeys. As in monkeys, the extent of the stripes shows the extent of V2. , The presence of stripes in human V2 suggests that it may have the same intricate functional and connectional organization as shown in other primates (see below).
Each of the CO stripes has a different set of connections ( Fig. 31.4 ). The thin stripes receive input from CO blob columns within V1, whereas the thick stripes and the pale-staining interstripes receive input from inter-blob columns. In each case, the projection originates from magnocellular-dominated layer 4B (layer 3C of Hassler; see Chapter 30 for the equation of Hassler’s and Brodmann’s layering schemata), layer 3B, which combines inputs from magno- and parvocellular channels (plus koniocelluar input in CO blob columns), and layer 3A, which has little or no direct input from LGN recipient layers. The corticocortically and sub-cortically projecting cells of V2 are also segregated into CO compartments. The thin stripes and interstripes project to V4, a ventral stream area involved in form processing, whereas the thick stripes project to MT, , a dorsal stream area associated with motion processing. Cells projecting sub-cortically to the superior colliculus are also preferentially clustered in the thick stripes. However, not all connections are segregated by stripe type. Feedback connections from higher-order visual areas tend not to be stripe specific and the local pathways within V2 interconnect stripes of all types. , Thus there is a substrate for binding different attributes of a visual stimulus into a coherent perceptual whole, even at relatively early levels of visual processing.

There are differences in the receptive field properties of cells in V2 that correlate with CO stripe type, although the absolute degree of segregation of receptive field types within and between CO compartments is debated. , Studies reporting segregation of single cell receptive field properties generally agree that thin stripes contain proportionately more cells with receptive fields that are unoriented and color opponent, whereas the interstripes contain more oriented cells and fewer color-coded units. Clustered in the thick stripes are cells that are oriented and sensitive for retinal disparity, which is important for generating depth perception. The clustering of properties within stripe types is thus consistent with the physiology of the areas to which they project.
The segregation of selectivity for orientation, color, and disparity within compartments of monkey V2 is supported by imaging experiments. There is one report of imaging experiments in human using high-resolution techniques to show that, within V2, stripes activated more strongly by slowly changing chromatic stimuli interleave with those activated by rapidly changing luminance stimuli, with a periodicity roughly equal to that of human V2 stripes. Within an individual CO compartment, there is a columnar organization according to preferred orientation , (thick and pale stripes), disparity (thick stripes), luminance (thin stripes), or color , (thin stripes), similar to maps of orientation preference in V1 (see Chapter 30 ).
The visual topography of V2 is interesting in that the CO stripes are oriented perpendicular to lines of isopolarity in V2, but parallel to the isoeccentricity lines. If V2 had a fine-grained continuous map of the visual field, sub-modalities of visual function associated with each stripe type would be restricted to parts of the visual field mapped onto that stripe. In fact, the visual map in V2 consists of three distinct, interleaved maps. , Each region of visual space is represented three times, once for each stripe type. The regular progression of receptive field positions seen when traversing across a single stripe are interrupted by jumps in receptive field location at stripe borders. This ensures that visual space is represented completely in all of the stripe types of V2.
Areas of the dorsal stream
The dorsal stream of visual processing deals with the general problem of where objects are in visual space and how to manipulate them. The dorsal stream solves two main problems, processing motion, and decoding position and 3-D features from 2-D retinal images. Dorsal stream-bound outputs from V1 and V2 funnel through MT and V3 to reach areas of the parietal cortex.
MT/V5 and related areas
A group of visual areas exists in the superior temporal sulcus (STS) of the macaque monkey, with likely homologs in other primate species, including humans. These areas are recognized by their interconnections and by the fact that many of their neurons are sensitive to the direction of motion of moving stimuli. The largest of these areas, V5, also known as MT (for Middle Temporal area), is probably the most easily identified visual area outside of V1. In addition to direction selectivity, MT is marked by heavy myelination and dark, patchy CO staining, a complete representation of the contralateral visual field, and strong direct input from V1 ( Box 31.3 ). In humans, MT corresponds to an area near the occipitotemporal junction, in the dorsal part of the inferior temporal sulcus, showing the dense myelination and dark, patchy CO staining seen in other primates. , Motion selectivity in human MT/V5 has been shown with imaging and evoked potential studies comparing activation with moving versus stationary stimuli; such studies also show human MT to have higher contrast sensitivity than surrounding cortex and to respond poorly to contours demarcated solely by wavelength.
- •
Extrastriate area of the dorsal (where) stream
- •
Displays motion, direction, and disparity sensitivity
- •
Strong direct inputs from layers 4B and 6 of V1
- •
Patchy CO staining and heavy myelination pattern
- •
Located in the occipitotemporal junction near the inferior temporal sulcus in humans
- •
Clinical condition of akinetopsia (loss of motion perception) develops after bilateral lesions to the lateral occipitotemporal cortex; unilateral lesions lead to a contralateral deficit that may go unnoticed by patients
MT receives projections from direction-selective cells in layers 4B (Hassler’s 3C) and 6 of both blob and interblob columns in V1 , and from the thick stripes of area V2. , As discussed previously ( Chapter 30 ), layer 4B is associated with the magnocellular pathway, while V2 thick stripes receive a mixture of both magnocellular and parvocellular inputs. Nevertheless, blocking the magnocellular layers of the LGN greatly affects the responses in MT, whereas blocking the parvocellular layers has much smaller effects on responses in MT, suggesting a dominance of the magnocellular pathway to MT. MT thus serves as a gateway for magnocellular information into other areas important for motion processing in the STS, the parietal lobe, and the frontal eye fields. Not surprisingly, lesions of MT in monkeys lead to deficits in motion processing, assessed behaviorally. Monkeys with lesions in MT have elevated detection thresholds of a motion signal in the midst of masking motion noise. The detection threshold for contrast of a visual stimulus was not affected by the MT lesion, showing that the MT lesion effect was selective for motion perception. Deficits for eye movements made to moving targets are also impaired by MT lesions ( Box 31.4 ), whereas eye movements made to stationary targets are unaffected by the MT lesions, suggesting that monkeys with MT lesions have more difficulty responding to moving than to stationary stimuli. Human patients with lesions that include MT have difficulty with motion perception, a condition known as akinetopsia. One such patient complained that she could not cross the street because of her inability to judge the speed of a car, although she could identify the car itself without difficulty, showing the specificity of the deficit. , Temporary inactivation of MT with TCMS causes a similar akinetopsia.
- •
Smooth pursuit involves motion processing in dorsal stream areas, MT, and MST
- •
Other cortical areas such as the frontal eye fields (FEF) and supplementary eye fields (SEF) contribute to the control of smooth pursuit
- •
Asymmetric smooth pursuit function indicates focal lesions in the lateral occipitotemporal (MT/MST) or dorsomedial frontal (FEF and SEF) regions (lesions limited to V1 do not impair smooth pursuit)
- •
Saccadic eye movements involve posterior parietal areas (PPC), the frontal eye fields (FEF), and the dorsolateral prefrontal cortex (DLPC)
- •
Parallel descending pathway from the FEF and SEF to the caudate nucleus, midbrain, and brainstem structures are also involved with saccadic eye movements
- •
Unilateral, focal cerebral lesions throughout frontal and parietal lobes may cause abnormalities of the in latency and accuracy of saccades
- •
Bilateral frontal or frontoparietal lesions may abolish saccades, sparing the quick phase of the vestibular ocular reflex (VOR), leading to acquired ocular motor apraxia
- •
Complete loss of all saccades, including reflexive saccades, quick phases, and smooth pursuit is called acquired supranuclear ocular motor apraxis and has been reported after small bilateral infarcts of both the posterior parietal cortex and FEF
Several forms of columnar organization are found within MT. These include organizations for direction tuning, disparity tuning, and selectivity for wide-field versus local motion contrast. The tangential organization of direction selectivity in MT resembles the organization of orientation selectivity in V1 (see Chapter 30 ); regions of gradual change in preference are interrupted by sudden shifts of approximately 180 degrees. Approximately 180 degrees of axis of motion are represented in 400 to 500 µm of cortex, similar to the size of orientation columns in V1. This organization of direction-selective neurons made possible an elegant experiment using cortical microstimulation in awake behaving monkeys, showing how the stimulus selectivity of neurons in visual cortex underlies the perception of visual stimuli. A microelectrode in MT in the middle of a cluster of neurons that shared a common preferred direction of motion was used to stimulate those neurons while the monkey performed a motion-discrimination task. Monkeys were required to discriminate between motion shown either in the direction preferred by the neurons or in the opposite direction, and noise was added to make the difficulty of discrimination near the monkeys’ threshold for being able to perform the task. When electrical micro-stimulation was applied, the monkeys indicated that the motion was in the stimulated neurons’ preferred direction more frequently than without stimulation. Thus a functional link was established between the activity of direction-selective neurons and perceptual judgments of motion direction.
In addition to motion selectivity, MT is also important for stereoscopic depth perception. Within MT, patches of disparity-selective neurons alternate with neurons not selective for disparity. Within a disparity-selective patch, there is a smooth progression of preferred disparity across the cortical surface and neurons in the same column have similar disparity tuning. The presence of disparity-tuned neurons in MT is not surprising, given the disparity tuning found in the thick stripes of V2 and the connections between the thick stripes and MT. Microstimulation experiments on disparity-tuned columns in MT similar to the previously described experiment for the direction columns show that electrical stimulation of clusters of disparity-selective MT neurons bias perceptual judgments of depth in a way that is predictable from the disparity preference of neurons at the stimulation site. Selectivity for stereoscopic depth has also been shown in human MT using fMRI. Thus MT is also important in the perception of depth, as well as motion. Moreover, the two certainly interact, as image movement is an important cue for depth perception.
V5 is strongly interconnected with three nearby motion-selective areas in the superior temporal sulcus, the lateral and dorsal divisions of the medial superior temporal areas (MSTl and MSTd), and area FST in the Fundus of the Superior Temporal sulcus. FST has a lower proportion of direction-selective cells than MT or MST, while MST has cells with larger receptive fields than in MT. MST can be divided into a lateral division preferring smaller stimuli and a dorsal division with cells responding to “optic flow” stimuli, i.e. rotation and expansion of objects, as would occur during movement through the environment. In humans, only a single visual area has been identified in this region, and it has been tentatively identified as MST, based on its large receptive fields and responses to optic flow stimuli. An area dorsal to MT in humans has been identified as being specialized for processing images of the human body, perhaps being involved in the analysis of biological motion.
V3
V3 consists of a narrow belt of cortex immediately adjacent to V2, with the representation of the horizontal meridian forming its border with V2, and the vertical meridian representation forming its outer border. The dorsal flank of V3 contains the lower visual field representation, and the ventral flank contains the upper field representation. Because the two parts of V3 appeared discontinuous across the representation of the horizontal meridian, being interrupted by V4, and because some studies also suggested functional and connectional differences between the two parts, the ventral flank was originally referred to as the ventral posterior area (VP) as distinguished from dorsal V3 (V3d), or V3 proper. ,
V3 has neurons selective for the basic stimulus parameters including orientation, color, direction, and binocular disparity. In both dorsal and ventral halves, cells preferring similar orientations are grouped into columns. , As in MT, cells with similar preferred disparity are also arranged in columns. About half the cells are strongly selective for direction of motion, more than any cortical area other than MT. , VP (ventral V3) has been reported to have more cells selective for color and fewer cells selective for direction.
V3 receives projections from V1 and V2 and is strongly interconnected with dorsal stream areas MT (and surrounding areas), and areas in the parietal lobe (PIP and VIP), although it also is connected with V4. Although it was originally thought that only dorsal V3 received projections from V1, connections between V1 and VP have been demonstrated. The V1 projections originate mostly from magnocellular-dominated layer 4B; the connections from V2 are discontinuous, suggesting they arise from a subset of V2 CO compartments, but it is not yet known which subset. V3 itself does not show a modular organization with CO staining, and it is not known how or if different classes of inputs from V1 and V2 are segregated within it.
V3 has been identified in human functional imaging studies, by its position relative to V2 and its similar visual field organization, , though it is not as narrow relative to V2 as it is in monkeys. Functional imaging studies show similar properties compared to monkey V3. Human V3 is sensitive to direction of motion and stereoscopic depth, but not as strongly as V3a, MT, and parietal areas. With regards to differences between the dorsal and ventral halves, these studies show little difference between V3d and VP in humans. Interestingly, VP did show, compared to dorsal V3, more activation during a color discrimination task relative to activation during a brightness discrimination task, consistent with earlier results in monkey V3. However, the same study showed a similar, though not as extensive, difference in relative activation of upper and lower visual field representations of V2 and V1. The consensus is thus emerging that V3d and VP are two parts of a single area, and that the differences between V3d and VP in monkeys are either not large enough to warrant division into two areas, or are due to mistakenly including adjoining areas in the analysis of VP due to its extreme narrowness in macaque monkeys.
V3A
V3A corresponds to a complete representation of the visual field located anterior to dorsal V3. , Although originally thought to function as an “accessory” to V3, this area is not especially tied to V3 functionally. In monkeys, the neurons of V3A differ from those of neighboring dorsal V3 in being less selective to the speed and direction of stimulus motion. , In humans, however, V3A is more motion sensitive than V3. Repetitive TCMS to V3A disrupts judgment of stimulus velocity as much as TCMS to MT. In both humans and monkeys, V3A is activated by disparity stimuli and responds better to achromatic stimuli than to color-defined stimuli, , placing it in the dorsal stream of visual processing. V3A receives ascending projections from V1, V2, and V3 and projects most strongly to other dorsal stream areas, including MT and MST and to parietal area LIP, an important area for coordinating stereoscopic vision with prehensile hand movements. Projections to temporal lobe areas exist, but are relatively minor.
PO/V6
PO and V6 were defined independently in macaque monkeys, but were later concluded to correspond, at least in part, to the same area in the parietal-occipital sulcus. V6 contains a complete representation of the visual field, with the representation of the horizontal meridian forming the posterior border, and the vertical meridian forming the anterior border. Peripheral visual field representation is enhanced in V6, relative to other areas. V6 receives an extensive, topographically organized, projection from V1, arising mostly from layer 4B, but with a component from layer 3B as well. As a dorsally located projection zone of V1, V6 resembles the medial area (M) and/or dorsal medial area (DM) described previously in New World monkeys (see Chapter 30 ). V6 is connected with other dorsal stream areas, and provides input to parietal cortex, especially cortex immediately anterior to it, know variously as V6A or MIP. An area claimed to correspond to V6 in humans was discovered in the posterior and superior part of the parieto-occipital sulcus, at the medial border of V3 and V3A. Large field stimuli were needed to generate responses in this area, as, similar to V6 in macaque monkeys, the map emphasizes the periphery of the visual field.
Parietal lobe areas
The dorsal stream of visual processing continues in a constellation of areas in the parietal lobe, particularly in the intraparietal sulcus, which form the interface between perceiving visual stimuli and planning motor actions for controlling arm and eye movements in space. In the macaque monkey, the most important of these are the lateral intraparietal area (LIP) and surrounding areas and area 7a. LIP receives input from MT and MST and, in turn, projects to area 7a. Area 7a sends ascending projections to frontal cortex, including the frontal eye fields, which are important in programming eye movements ( Box 31.4 ), as well as cingulate and parahippocampal cortices. Area 7a is also interconnected with subcortical structures related to ocular and vestibulo-ocular function.
Receptive fields of intraparietal areas are large, as much as 60 degrees across, and often extend across the midline. Different classes of intraparietal neurons have been identified with sensitivities for: depth relative to the fixation plane, linear movement in depth, rotation in depth, 3D orientation for object manipulation, and 3D axis or surface orientation. Neurons in area 7a also receive extra-retinal oculomotor inputs, and their firing may be modulated by the horizontal and vertical position of the eyes in the orbits. The combined eye position and visual signal in area 7a is sufficient to determine the spatial location of an object. Accordingly, lesions in this area lead to deficits in reaching for an object in three dimensions using visual guidance.
As in monkeys, the intraparietal sulcus in humans is important for visuomotor tasks comprising target selections for arm and eye movements, object manipulation, and visuospatial attention. At least four intraparietal visual areas have been identified in humans, , although the homology of each of these areas with individual areas in macaque monkeys is unresolved, probably reflecting true species differences. , Lesions of parietal visual areas in humans lead to apraxia, neglect, and Balint’s syndrome. Similarly, TCMS of human posterior parietal cortex degrades accuracy of saccades to remembered locations, , disrupts visually guided reaching behavior, and reduces detection and exploration of contralateral stimuli.
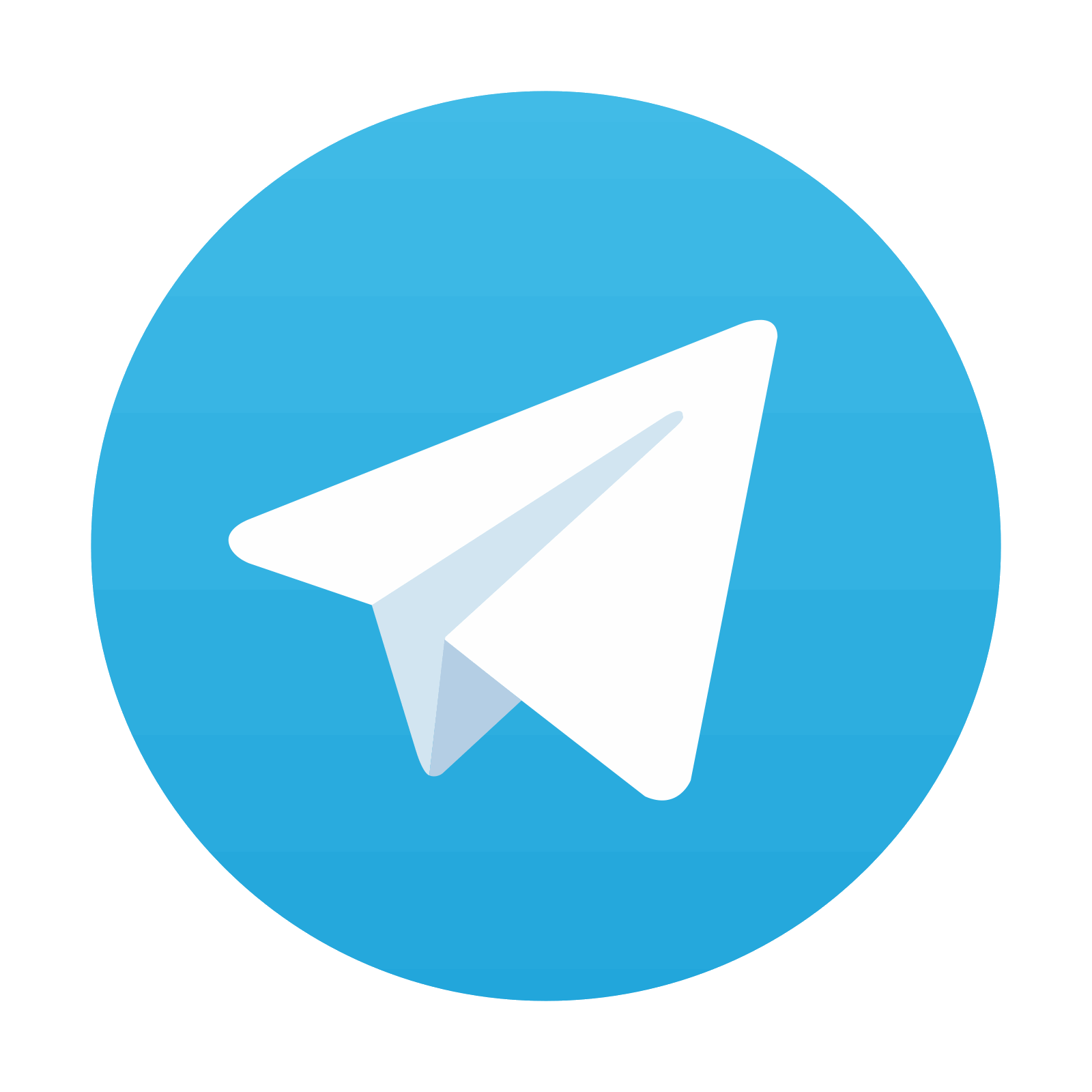
Stay updated, free articles. Join our Telegram channel
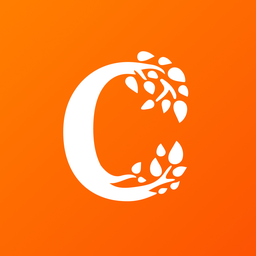
Full access? Get Clinical Tree
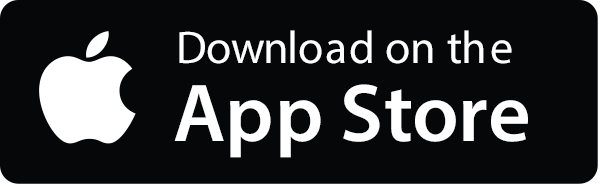
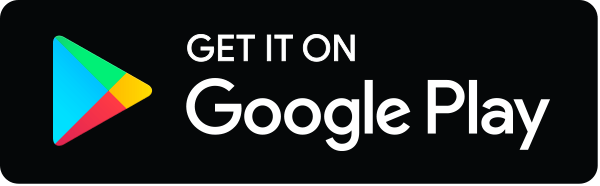