Introduction
The electroretinogram (ERG) is a useful tool for objective, non-invasive assessment of retinal function both in the clinic and the laboratory. It is a mass electrical potential that represents the summed response of all the cells in the retina to a change in illumination. Recordings can be made in vivo under physiological or nearly physiological conditions using electrodes placed on the corneal surface. For standard recordings in the clinic, ERGs such as those illustrated in Figure 24.1 are recorded from alert subjects who are asked not to blink or to move their eyes. Anesthesia, selected for having minimal effects on retinal function, may be required for recordings from some very young human subjects, and is generally used for recordings in animals. The positive and negative waves of the ERG reflect the summed activity of overlapping positive and negative component potentials that originate from different stages of retinal processing. The choice of stimulus conditions and method of analysis will determine which of the various retinal cells and circuits are generating the response. Information about retinal function provided by the ERG is useful for diagnosing and characterizing retinal diseases, as well as for monitoring disease progression, and evaluating the effectiveness of therapeutic interventions.

Much of the basic research on the origins, pathophysiology and treatment of retinal diseases that occur in humans is carried out in animal models. The ERG provides a simple objective approach for assessing retinal function in animals. In recent years it has been of particular benefit in studies of mouse (rat, and other species) models of retinal disease, and it has been valuable for evaluating the retinal drug toxicity. The ERG also has been useful for characterizing changes in retinal function that occur as a consequence of genetic alterations in mice and other models, which affect the transmission and processing of visual signals in the retina.
This chapter provides information on retinal origins and interpretation of the ERG, with emphasis on advances in our understanding of the ERG that have occurred through pharmacological dissection studies in macaque monkeys whose retinas are similar to those of humans. The similarity of the waveforms of flash ERGs of humans and macaques can be seen in Figure 24.2 . The chapter also will examine origins of the mouse ERG, and consider similarities and differences between mouse and primate ERG. Although the focus of this chapter is on primate and rodent retinas, it is important to note that the ERG is a valuable tool for assessing retinal function of all classes of vertebrates. This includes amphibians and fish in which classical studies of the retina were carried out (for a review, see Dowling ) and in which current work continues to improve our understanding of retinal function under normal, genetically altered and pathological conditions.

This chapter provides useful background information for interpreting the ERG, but does not provide a comprehensive review of the characteristics of ERGs associated with retinal disorders encountered in the clinic. Recent texts by Fishman et al, Heckenlively & Arden and Lam are recommended resources for learning more about clinical applications.
Generation of the ERG
Radial current flow
The ERG is an extracellular potential that arises from currents that flow through the retina as a result of neuronal signaling, and for some slower ERG waves, potassium (K + ) currents in glial cells. Local changes in the membrane conductance of activated cells give rise to inward or outward ion currents, and cause currents to flow in the extracellular space (ECS) around the cells, creating extracellular potentials. Although all retinal cell types can contribute to ERGs recorded at the cornea, the contribution of a particular type may be large, or hardly noticeable in the recorded waveform, depending upon several factors detailed below.
The orientation of a cell type in the retina is a major factor in determining the extent to which its activity will contribute to the ERG. A schematic drawing of the mammalian retina, with the various cell types labeled, can be found in Chapter 21 ( Fig 21.1 , Fig 21.2 ). When activated synchronously by a change in illumination, retinal neurons that are radially oriented with respect to the cornea, i.e. the photoreceptors, and bipolar cells, make larger contributions to the major waves of the ERG than the more laterally oriented cells and their processes, i.e. the horizontal and amacrine cells. The major waves at light onset are, as marked in Figures 24.1 and 24.2 , an initial negative-going a-wave (mainly from photoreceptors), which is truncated by a positive-going b-wave, mainly generated by ON (depolarizing) bipolar cells. For longer duration flashes ( Fig. 24.2 , bottom row), the light-adapted ERG includes another positive-going wave, the d-wave, at light offset, with major contribution from OFF (hyperpolarizing) bipolar cells. Currents that leave retinal cells and enter the ECS at one retinal depth (the current source), will leave the ECS to re-enter the cells at another (the current sink), creating a current dipole. These retinal currents also travel through the vitreous humor to the cornea, where the ERG can be recorded non-invasively, as well as through the extraocular tissue, sclera, choroid and high resistance of the retinal pigment epithelium (RPE) before returning to the retina. Local ERGs can be recorded near the retinal generators using intraretinal microelectrodes in animals, while simultaneously recording the global ERG elsewhere in the current path, e.g. from the corneal surface, or using an electrode in the vitreous humor, with a reference electrode behind the eye. Such recordings have provided useful information about the origins of the various waves of the ERG.
Glial currents
The ERG waveform also will be affected by glial cell currents. Retinal glial cells include Müller cells, RPE cells, and radial astrocytes in the optic nerve head. One crucial function of glia is to regulate extracellular K + concentration, [K + ] o , to maintain the electrochemical gradients across cell membranes that are necessary for normal neuronal function. Membrane depolarization and spiking in retinal neurons that occur in response to changes in illumination lead to leak of K + from the neurons and to K + accumulation in the ECS. Membrane hyperpolarization, in contrast, leads to lower [K + ] o as the membrane leak conductance is reduced, but the Na + K + ATPase in the membrane continues to transport K + into the cell.
K + currents in Müller cells move excess K + from areas of high [K + ] o to areas of lower [K + ] o by a process called spatial buffering. Return currents in the retina are formed by Na + and Cl – . The regional distribution and electrical properties of inward rectifying K + (Kir) channels in Müller cells (see Fig. 24.3C ) are critical for the spatial buffering capacity of the cells. Studies of Kofuji and co-workers have shown that strongly rectifying Kir2.1 channels in Müller cells are located in ‘source’ areas, particularly in synaptic regions where [K + ] o is elevated due to local neuronal activity. In contrast, Kir4.1 channels (weakly rectifying) are densest in Müller cell endfeet, in inner and outer limiting membranes and in processes around blood vessels. K + enters Müller cells through Kir2.1 channels that minimize K + outflow and K + exits the Müller cells via the more bidirectional Kir4.1 channels to enter the extracellular ‘sink’ areas with low [K + ] o : the vitreous humor, subretinal space (SRS), and blood vessels.

ERG waves associated with glial K + currents have a slower timecourse than waves related to currents around the neurons whose activity causes the changes in [K + ] o . As [K + ] o increases or decreases with neuronal activity, the resulting glial K + currents will be related to the integral of the K + flow rate, e.g. the glial contribution to the b-wave modeled in Figure 24.11 . Other waves generated by glial K + currents in Müller cells, or other retina cells with glial function, such as the RPE, include the c-wave and slow PIII ( Fig. 24.3A,B ), both related to the reduction in [K + ] o in the SRS that occurs when photoreceptors hyperpolarize in response to a strong flash of light. The negative scotopic threshold response (nSTR) and photopic negative response (PhNR), both originating from inner retinal activity, are also thought to be mediated by glial K + currents in retina or optic nerve head.
Stimulus conditions
Aside from structural and functional aspects of the retina, stimulus conditions are of great importance in determining the extent to which particular retinal cell or circuits contribute to the ERG. Signals will be generated in rod pathways, in cone pathways or both depending upon the stimulus energy, wavelength and temporal characteristics, as well as upon the extent of background illumination, with rods responding to, and being desensitized by lower light levels than cones. Fully dark-adapted, ERGs driven by rods only (i.e. scotopic ERGs) are thus useful for assessing rod pathway function ( Figs 24.1 and 24.2 , top), and light-adapted ERGs driven only by cones (i.e. photopic ERGs), for assessing cone pathway function ( Figs 24.1 and 24.2 , bottom). Figure 24.1 , bottom right, also shows responses to 30 Hz flicker which isolates cone-driven responses because rod circuits do not resolve well such high frequencies. Very bright light flashes elicit small wavelets superimposed on the b-wave called oscillatory potentials (OPs) that are generated by circuits proximal to bipolar cells. OPs can be isolated by bandpass filtering: 75–300 Hz in Figure 24.1 , top right.
The spatial extent of the stimulus is an important factor in ERG testing. For standard clinical tests, as illustrated in Figure 24.1 , and listed in Box 24.1A , as well as for most testing of animal models, a full-field (Ganzfeld) flash of light is used ( Fig. 24.2B ). A full-field stimulus generally elicits the largest responses because more retinal cells are activated and the extracellular current is larger than for focal stimuli. It also has the advantage that all regions of retina are evenly illuminated, and with respect to background illumination, evenly adapted. Pupils are generally dilated for full-field stimulation. More spatially localized (focal) stimuli are useful for analysis of function of particular retinal regions, e.g. foveal vs peripheral regions in primates. Multifocal stimulation allows assessment of many small regions simultaneously.
A
Standard ERG tests
Described by ISCEV Standard for full-field clinical Electroretinography (2008 update). All numbers are stimulus calibrations in cd.s.m –2
- •
Dark-adapted 0.01 ERG (“rod response”)
- •
Dark-adapted 3.0 ERG (“maximal or standard combined rod–cone response”)
- •
Dark-adapted 3.0 oscillatory potentials (“oscillatory potentials”)
- •
Light-adapted 3.0 ERG (“single-flash cone response”)
- •
Light-adapted 3.0 flicker ERG (“30 Hz flicker”)
- •
Recommended additional response: either dark-adapted 10.0 ERG or dark-adapted 30.0 ERG
B
Specialized types of ERG and recording procedures
- •
Macular or focal ERG
- •
Multifocal ERG (see published guidelines )
- •
Pattern ERG (see published standard )
- •
Early receptor potential (ERP)
- •
Scotopic threshold response (STR), negative and positive
- •
Photopic negative response (PhNR)
- •
Direct-current (dc) ERG
- •
Electro-oculogram (see published standard )
- •
Long-duration light-adapted ERG (ON–OFF responses)
- •
Paired-flash ERG
- •
Chromatic stimulus ERG (including S-cone ERG)
- •
Dark and light adaptation of the ERG
- •
Dark-adapted and light-adapted luminance-response analyses
- •
Saturated a-wave slope analysis
- •
Specialized procedures for young and premature infants
ERG responses illustrated in Figure 24.1 are to a minimum set of stimuli selected by the International Society for the Clinical Electrophysiology of Vision (ISCEV) to efficiently acquire standard, comparable data on rod and cone pathway function from clinics and laboratories around the world. Names of the standard tests are listed in Box 24.1A . Tests using stimuli presented over a fuller range of stimulus conditions to allow more complete or specific evaluation of retinal function, are listed in Box 24.1B , and a few of these tests will be described later in this chapter.
Non-invasive recording of the ERG
ERGs can be recorded from the corneal surface using various types of electrodes. A commonly used electrode, with good signal-to-noise characteristics, is a contact lens with a conductive metal electrode set into it (Burian Allen electrode). It has a lid speculum to reduce effects of blinking and eye closure. In the bipolar form of the electrode, the outer surface of the lid speculum is coated with conductive material that serves as the reference. This type of electrode is best tolerated (in alert subjects) when a topical anesthetic is used. Other types of contact lens electrodes have been used as well, e.g. the jet electrode which is disposable. Some clinicians and researchers use thin mylar fibers impregnated with silver particles, called DTL electrodes, as illustrated in Figure 24.2B , or gold foil, or wire loop electrodes (H-K loop) that hook over the lower eyelid. For rodents, metal wires, in loops or other configurations placed in contact with the corneal surface are often used. Some labs use cotton wick electrodes and some use DTL fibers under contact lenses, or another form of contact lens electrode. Corneas are kept hydrated with a lubricating conductive solution in all cases. The reference electrode can be placed under the ey lid, e.g. the speculum of a contact lens electrode, as described above, or remotely, for example on the temple, the forehead, or the cornea of the fellow eye. ERG signals ranging from > microvolt to a millivolt or more, peak to peak, for responses to strong stimuli, are amplified, and digitized for computer averaging and analysis. Filtering is done to remove signals outside the frequency range of retinal responses to stimulation (<1 and >300 Hz), and to remove line frequency noise (e.g. 50 or 60 Hz).
Classical definition of components of the ERG
The origins of the various waves of the ERG have been of longstanding interest to clinicians and researchers. Our current understanding of the cellular origins of the ERG profits from extensive knowledge, as described in previous chapters, of the functional microcircuitry of the retina, and particularly of the physiology and cell biology of the retinal cell types, and the identity and action of retinal neurotransmitters, their receptors, transporters and release mechanisms. However, a classical study, using ether anesthesia, provided the first pharmacological separation of ERG components.
Granit’s classical pharmacological dissection of the ERG, illustrated in Figure 24.4 , provided valuable insights on origins of ERG waves as well as a nomenclature for waves based on their distinct retinal origins. Component “processes” were found to disappear from the ERG during the induction of ether anesthesia in the following order: process (P)I, the slow c-wave response that follows the b-wave; generated predominantly by the RPE; PII, the b-wave, generated by bipolar cells; and eventually PIII, the photoreceptor-related responses that remained the longest. PII and PIII are still commonly used terms for ERG components generated by ON bipolar cells and photoreceptors respectively.

Slow PIII, the c-wave and other slow components of the direct current (dc)-ERG
PIII of the ERG can be separated into a fast and a slow portion: fast PIII is the a-wave, which reflects photoreceptor current (see below), and slow PIII results from Müller cell currents induced by photoreceptor-dependent reduction in [K + ] o in the SRS ( Fig. 24.3C ). Negative-going slow PIII and the positive-going pigment epithelial response to the same reduction in subretinal [K + ] o add together to form the c-wave, which, as shown in Figures 24.3 and 24.4 , is positive-going in the dark-adapted ERG of the cat. This is because, as illustrated by the intraretinal recordings from intact cat eye in Figure 24.3A , the positive-going RPE contribution, is larger than the negative-going Müller cell contribution. In mice, the c-wave also is positive-going, as shown in Figure 24.3B . In humans and monkeys, slow PIII and the RPE c-wave are more equal in amplitude, and the corneal c-wave is less positive. Two slower potentials that arise from the RPE, the fast oscillation potential (FO) and light peak (LP), are also present in cat and mouse dc-ERG recordings ( Fig. 24.3 ). The mouse LP is much smaller in amplitude than that in the cat. The cellular mechanisms that generate these slow waves were reviewed in more detail in previous reviews of ERG origins.
In alert human subjects it is not possible to obtain stable dc-ERG recordings necessary for recording slow events, e.g. those arising from the RPE, that occur over seconds or minutes, because the eyes move too frequently. Therefore, to measure slow potentials, the electro-oculogram (EOG), an eye-movement-dependent voltage, is recorded. The EOG is a corneo-fundal potential that originates largely from the RPE; its amplitude changes with illumination, being maximal at the peak of the light peak. Use of EOGs to evaluate retinal/RPE function is described in an ISCEV Standard publication on clinical EOG.
Full-field dark-adapted (Ganzfeld) flash ERG
Figure 24.5 shows full-field dark-adapted ERG responses to a range of stimulus strengths for a human subject (left), a macaque monkey, whose retina and ERG are similar to that of human (middle), and a C57BL/6 mouse (right). The mouse ERG is similar to the primate ERG, but larger in amplitude (see calibrations). For higher stimulus strengths than shown in the figure, the mouse ERG develops larger oscillatory potentials than those generally seen in the primate ERGs (e.g. Fig. 24.6 ). The ERGs in Figure 24.5 were generated almost entirely, except for responses to the strongest stimuli, by the most sensitive, primary rod circuit, This circuit is described more fully in previous chapters. For all three subjects, the strongest stimuli evoked an a-wave, followed by a b-wave. For stimuli more than two log units weaker than the strongest one, b-waves were still present, but a-waves were no longer visible. B-waves can be seen in responses to weaker stimuli than a-waves partly because of the convergence of many rods (20–40) onto the rod bipolar cells generating the response, which increases their sensitivity, and partly because of the large radial extent of ON bipolar cells in the retina. The slow negative wave in the ERGs of the three subjects in response to the weakest stimuli, called the (negative) scotopic threshold response (nSTR), and the equally sensitive positive (p)STR are related to amacrine and/or ganglion cell activity, as described more fully in a later section. The high sensitivity of the STRs relative to b-waves (and a-waves) reflects the additional convergence of rod signals in the primary rod circuit in the inner retina proximal to the rod bipolar cells.



Dark-adapted a-wave
It has long been appreciated that the dark-adapted a-wave primarily reflects the rod receptor photocurrent. The a-wave generator was localized to photoreceptors in classical intraretinal recording studies in mammalian retinas, some of which included current source density (CSD), or source-sink, analyses. The most direct demonstration of the a-wave’s cellular origin was provided in such experiments by Penn and Hagins in isolated rat retina. These experiments produced evidence that light suppressed the circulating (dark) current of the photoreceptors, and the investigators proposed that this suppression is seen in the ERG as the a-wave.
Negative ERGs
The receptoral origin of the a-wave also was demonstrated in early studies in amphibians using compounds that blocked synaptic transmission, Mg 2+ , Co 2+ , and Na + -aspartate, and isolated photoreceptor signals in the ERG, while abolishing responses of postreceptoral neurons. These manipulations also caused the b-wave to disappear, indicating its postreceptoral origin. As our understanding of synaptic pharmacology has improved, it has become more common to use glutamate agonists and antagonists to block transfer of signals from photoreceptors to specific second-order neurons. For example, blocking metabotropic transmission to depolarizing (ON) bipolar cells, with l -2-amino-4-phosphonobutyric acid (APB or AP4) an mGluR6 receptor agonist, eliminates the b-wave, and produces a negative ERG. Much of the remaining ERG is the rod photoreceptor response, although late negative signals also arise from OFF pathway neurons.
An essentially identical negative ERG to that after APB occurs in mice in which the mGluR6 receptor is genetically deleted, or when there are mutations in the mGluR6 receptor or other proteins whose function is necessary for normal signal transduction in ON bipolar cells. For example, Figure 24.6 shows the typical negative ERG of the dark-adapted Nob1 , i.e. no b-wave, mouse. The Nob1 mouse has a mutation in the Nyx gene that encodes nyctalopin, a protein found in ON bipolar cell dendrites. Mutation of this protein produces a negative ERG in human patients who are diagnosed with X-linked complete congenital stationary night blindness (CNSB-1). Negative ERGs also occur for other forms of CSNB caused by mutations in mGlur6 receptors, and in Nob3 and Nob4 mice with such mutations, as well as in Nob2 mice in which glutamatergic transmission from photoreceptors is compromised. Although the ERG of the Nob1 , and other mice lacking b-waves, is almost entirely negative-going, it rises from its trough at the timecourse of the c-wave. Photoreceptor-dependent slow responses such as c-wave and slow PIII are not affected by blockade of postreceptoral responses in neural retina.
Retinal ischemia due to compromised inner retina circulation also isolates the a-wave and eliminates postreceptoral ERG components. This was demonstrated in early experiments in monkeys by occlusion of the central retinal artery. A “negative ERG” in which b-waves are reduced or missing is a common clinical readout of central retinal artery and vein occlusions, as well as other disorders affecting postreceptoral retina such as melanoma-associated retinopathy, X-linked retinoschisis, muscular dystrophy or toxic conditions.
Modeling
The utility of the a-wave in studies of normal and abnormal photoreceptor function was advanced by the development of quantitative models based on single cell physiology that could predict the behavior both of the isolated photoreceptor cells and ERG a-wave in the same or similar species. Hood and Birch demonstrated that the behavior of the leading edge of the dark-adapted a-wave in the human ERG can be predicted by a model of photoreceptor function derived to describe in vitro suction electrode recordings of currents around the outer segments of single primate rod photoreceptors. Lamb and Pugh followed a simplified kinetic model of the leading edge of the photoreceptor response (in vitro current recordings initially in amphibians) that took account of the stages of the biochemical phototransduction cascade in vertebrate rods (see Chapter 21 ). This model was subsequently shown to predict the leading edge of the human dark-adapted a-wave generated by strong stimuli, and has been used extensively in clinical studies of retinal disease, and in analyses of photoreceptor function in animal models. A simplified formulation presented by Hood and Birch often is used (see legend to Fig. 24.7 ), and can be adjusted to application to cone signals as well. Figure 24.7 shows fits of Hood and Birch’s model to the dark-adapted a-wave of a normal human subject and a patient with retinitis pigmentosa (RP).

R ( I , t ) = ( 1 − exp [ − I * S * ( t − t d ) 2 ] ) * R m a x For t > t d
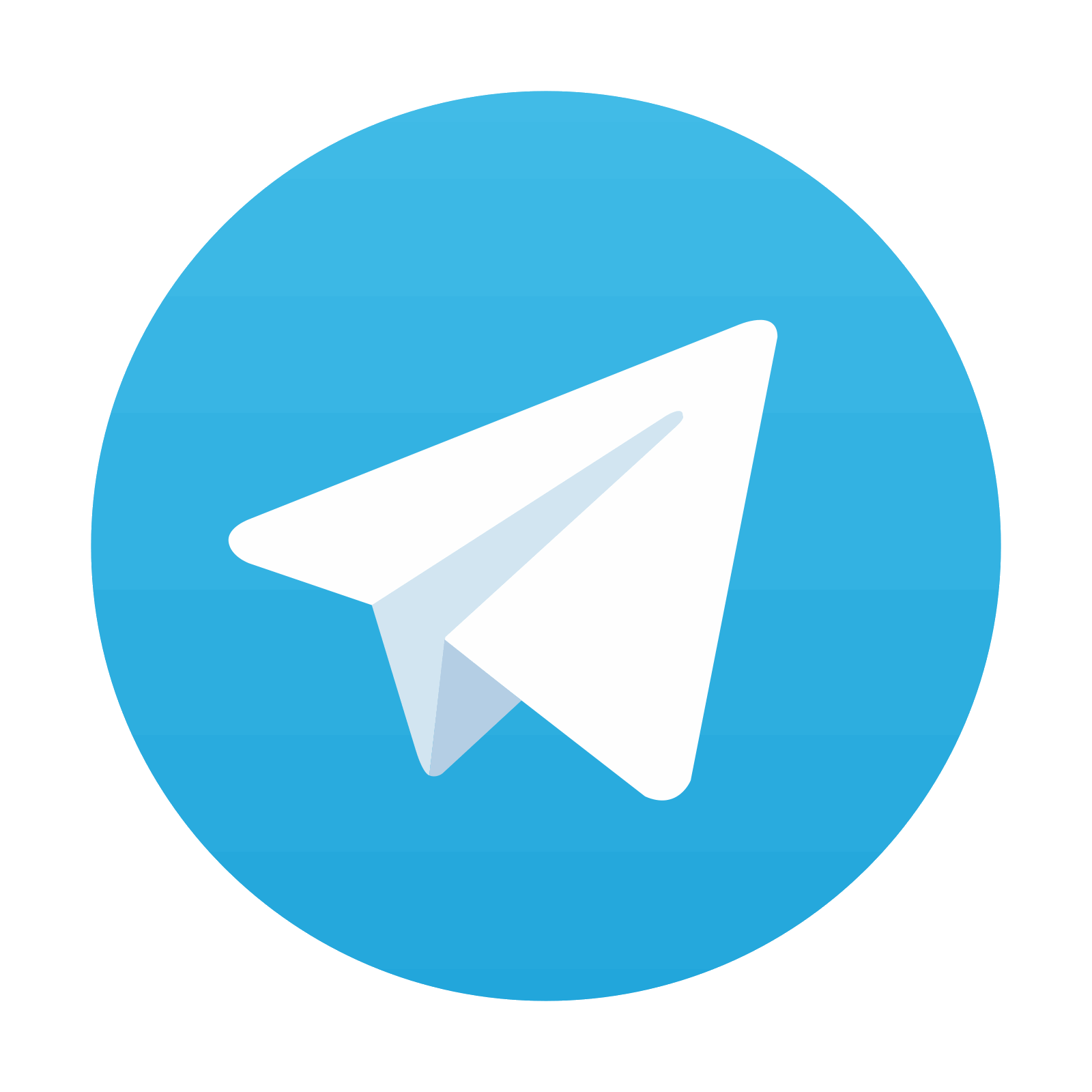
Stay updated, free articles. Join our Telegram channel
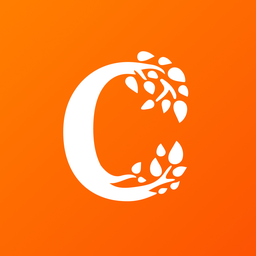
Full access? Get Clinical Tree
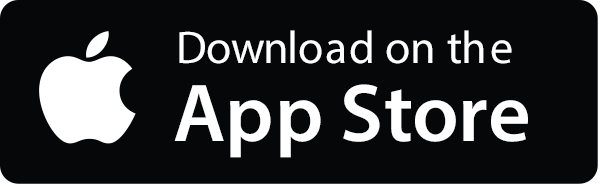
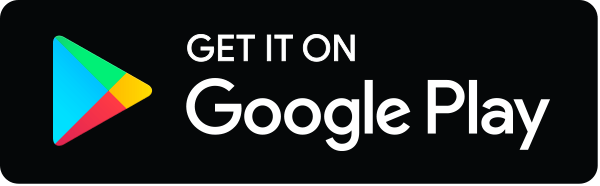
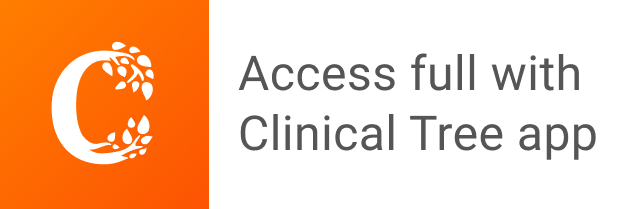