The vision of newborn infants is crude. As infants experience the normal visual environment, their vision rapidly improves with different visual capabilities emerging at different ages. Determining the exact timing for the behavioral onset of specific visual functions and identifying the critical factors that limit their development have been the primary focus of perceptual and physiological studies on vision development. Although immaturities of the physiological optics and ocular motility are known to affect the infant’s vision soon after birth, the maturation of the retina and, to a greater extent, the visual cortex largely set a limit on their normal perceptual development. ,
The neuronal connections of the visual cortex are malleable for a considerable time after birth, the “critical” or “plastic” period of development. The postnatal development of the visual cortex, therefore, requires normal visual experience and precise matching of the images in the two eyes. Experiencing binocularly discordant images early in life, a binocular imbalance, has devastating effects on the development of the visual system because after eye opening, the neurons in the highly plastic visual cortex receive robust signals from the two eyes that do not match. Prevalent abnormal visual conditions that can cause binocular imbalance are early monocular form deprivation, unilateral defocus, and ocular misalignment. Experiencing binocular imbalance during early infancy causes binocular vision disorders, and if untreated, amblyopia is likely to develop.
Topics in this chapter cover what we currently know about the perceptual consequences of early abnormal visual experience, the neural basis of altered vision, and the synaptic and molecular mechanisms of cortical plasticity.
Macaque monkeys are ideal animal models for exploring the neural mechanisms underlying developmental vision disorders in humans. The anatomical and physiological organizations of their visual system are nearly identical to humans. Perceptual studies in normal mature monkeys have extensively documented the striking similarities in monocular and binocular visual capabilities between macaque monkeys and humans. , The relative (scaled) time course of normal visual development in macaque monkeys parallels that of humans. The primate visual cortex is structurally and functionally , more developed at or near birth than the visual cortex in lower species.
Many important discoveries on vision development have been made on sub-primate species (e.g. cats, ferrets, rats, and mice). However, in lower animals it is not always possible to establish a link between neural and perceptual deficits resulting from early abnormal visual experience. This chapter, therefore, will primarily review studies on non-human primates. Studies with human infants are mentioned when appropriate, and research in sub-primate species is described in detail mostly where the neural and molecular basis of cortical plasticity is discussed.
Effects of early monocular form deprivation
Constant monocular form deprivation
Monocular form deprivation can result from an occlusion of the image in one eye or from a severely degraded image in the affected eye. Congenital dense cataracts and ptosis are the common causes of monocular form deprivation in human infants. To create primate models of monocular form deprivation, the eyelids of infant monkeys are surgically closed, or more recently, by wearing diffuser lenses in front of one eye.
Perceptual deficits
All binocular functions including local/global stereopsis and binocular summation of contrast sensitivity are severely compromised or lost following early monocular form deprivation. , The visual sensitivity of the deprived eye is dramatically reduced or virtually lost, form deprivation amblyopia ( Fig. 40.1A ). Importantly, the severity of the contrast sensitivity loss resulting from monocular form deprivation is directly related to the degree of retinal image degradation during early infancy ( Fig. 40.1B ).

Monocular deprivation also leads to an abnormal elongation of the eyes, hence, the development of myopic refractive errors. The deleterious effects of early monocular deprivation on spatial vision development are generally far more severe than the anomalies resulting from form deprivation in both eyes, bilateral form deprivation ( Fig. 40.2 ). , As in monkeys with monocular form deprivation, bilateral form deprivation leads to a significant loss of binocular functions including the detection of stereoscopic cues and binocular summation of contrast sensitivity ( Fig. 40.2 ). In these binocularly deprived monkeys, the binocular contrast sensitivity function (square symbols) overlaps with the better monocular sensitivity function (open circles).

Neural changes
The most consistent effect of early monocular form deprivation on development is anomalous changes in the ocular dominance distribution of V1 neurons ( ocular dominance plasticity ). During the critical period of development, the afferent fibers from the LGN representing the two eyes compete for consolidation of functional connections in V1 ( binocular competition ). , This early binocular competition is activity dependent; hence, depriving normal signals from one eye puts the affected eye into a competitive disadvantage and leads to a severe loss of functional connections in V1 from the deprived eye. The ocular dominance columns of the input layer in V1 (layer IVC) representing the deprived eye exhibit a substantial shrinkage. The axon arbors of the afferent fibers from the LGN in the deprived columns show abnormal structural changes and the intrinsic long-range horizontal connections extending over multiple ocular dominance columns reorganize their wiring pattern in the cat primary visual cortex. ,
Electrophysiological studies consistently report the severe loss of binocularly driven cells, i.e. neurons that can be activated by stimulation of either eye. In addition, there is a clear shift in the ocular dominance distribution of cortical neurons away from the deprived eye. Specifically, the percentage of V1 neurons that can be activated or dominated by stimulation of the deprived eye is significantly decreased ( Fig. 40.3 ). The reduced functional innervation from the deprived eye is, at least in part, the neural basis for “undersampling” of visual scenes by the affected eye in form deprivation amblyopia.

For subcortical structures, there is a mild shrinkage of cell bodies of LGN neurons that receive input signals from the deprived eye. However, the response properties of these primate LGN neurons are largely unaffected by early monocular form deprivation. Interestingly, there is considerable evidence for functional alterations in the cat LGN due to early abnormal visual experience. For the primate retina there are no significant structural or functional abnormalities due to early monocular form deprivation. Together, major neural changes resulting from early monocular form deprivations in primates occur beyond the LGN, i.e. begins in the primary visual cortex.
Intermittent monocular deprivation
Experiencing “normal vision” during early monocular form deprivation ( intermittent monocular form deprivation ) reduces some of the deleterious effects of early constant monocular form deprivation. The effects of early intermittent deprivations have been studied using different rearing regimens including daily alternating monocular deprivation, reverse occlusion, and monocular form deprivation with daily brief periods of unrestricted vision.
Alternating monocular deprivation
Daily alternations of form deprivation between the two eyes have very little impact on the perceptual development of either eye in cats. , Consistent with this observation, the spatial receptive-field properties such as orientation selectivity are normal. However, the same daily alternating deprivation devastates the development of binocular vision. Local stereopsis is lost and the proportion of binocularly driven neurons in area 17 is severely reduced. In monkeys, daily alternating monocular occlusion beginning at birth leads to a variety of abnormal eye positions and eye movements including strabismus and/or saccadic disconjugacy, i.e. the amplitudes of saccades in the occluded eye are less than that in the viewing eye. ,
Reverse occlusion
The effects of constant form deprivation in one eye, including spatial contrast sensitivity loss and ocular dominance shift in V1 away from the deprived eye, can be reversed if vision of the originally deprived eye is restored early in development and the fellow non-deprived eye is occluded, reverse occlusion , ( Fig. 40.4 ). The timing of the reverse occlusion is critical in determining the effectiveness of this procedure because the “recovery” of functions in the originally deprived eye may occur at the expense of the originally non-deprived eye. For example, contrast sensitivity can be restored if reverse occlusion occurs relatively early in the critical period, i.e. if the original deprivation is short. However, this early reversal leads to a loss of contrast sensitivity in the newly deprived (or originally non-deprived) eye (red circles in Fig. 40.4A ) and causes a corresponding shift in the ocular dominance distribution of V1 neurons favoring the initially deprived eye. There is an optimal time for the reversal of monocular occlusion in order to achieve near normal contrast sensitivity for both eyes ( Fig. 40.4B ). , In all cases, the binocular functions are diminished. Similar effects of reverse occlusion have been extensively studied in cats, and the results have contributed in advancing our understanding of the neural mechanisms underlying the breakdown and recovery of visual functions from early monocular form deprivation.

The clinical significance of these findings is that this kind of animal study can provide key information for developing an effective clinical strategy for treating amblyopia with various patching regimens .
Brief unrestricted vision during monocular deprivation
Providing brief daily periods of normal vision to the deprived eye during early monocular deprivation prevents or reduces the severity of form deprivation amblyopia in monkeys ( Fig. 40.5A ). Constant form deprivation (0 hour of unrestricted vision), as previously described, causes severe amblyopia of the deprived eye and a large shift in the ocular dominance of V1 neurons away from the deprived eye ( Fig. 40.5B ). However, only one hour of unrestricted (normal) vision every day during the deprivation period (12 hours/day) dramatically improves the contrast sensitivity of the deprived eye, hence reducing the severity of form deprivation amblyopia. In these monkeys the extent of abnormal ocular dominance shift in V1 is significantly reduced ( Fig. 40.5B ). In stark contrast, the same “preventive” measure, even with 4 hours of daily unrestricted vision during the 12-hour deprivation period, does not prevent a severe loss of disparity sensitive neurons, highlighting the extremely fragile nature of developing binocular connections in V1. However, one hour of unrestricted vision is effective in reducing binocular suppression in V1 (i.e. the binocular responses of V1 neurons are less than monocular responses) that is prevalent in monkeys reared with constant form deprivation ( Fig. 40.5C ).

Constant monocular form deprivation leads to an elongation of the eye, and hence, the development of myopic refractive errors in the deprived eye. However, a brief period of unrestricted vision during the deprivation period reduces the degree of myopic refractive error.
The clinical relevance of these studies is that the timely removal of the conditions that produce degradation of images or image occlusion (e.g. severe hyperopic anisometropia, cataract, or ptosis) is critically important for the prevention of amblyopia. If that is not immediately possible, stop-gap manipulations such as lifting a drooping eyelid or keeping corrective lenses even for a short period of time every day are likely to have preventive effects against form deprivation amblyopia and development of myopic refractive errors. Taken together, studies of this kind and those on the effects of temporal variations in image quality, e.g. alternating or reverse occlusion, can provide key information in developing effective clinical strategies for the management of amblyopia in human infants. ,
Critical period
The critical (plastic) period of vision development is traditionally defined as the postnatal period during which visual deprivation leads to long-term or permanent structural and/or functional changes of the visual system. The critical period differs substantially between species, the visual functions affected by deprivation, sites of neural alterations, and the nature of the visual deprivation, e.g. dark rearing, monocular form deprivation, monocular defocus, or ocular misalignment. For example, the critical period for primates, unlike sub-primate species, begins at or near birth ( Fig. 40.6 ). Binocular functions are generally more readily disrupted by early visual deprivations than monocular spatial vision. The critical period for experience-dependent changes differs between cortical sites, e.g. V1, V2, V4 or MT, and cortical layers within a given cortical site. The higher stages of processing, e.g. supra- and infra-granular layers compared to input layer within V1 or cortical sites later in the hierarchy of extrastriate visual areas, appear to have longer periods of plasticity.

Critical period for monocular form deprivation
There are multiple “plastic” periods for different visual functions in macaque monkeys ( Fig. 40.6 ). , Spectral sensitivity functions have relatively short critical periods that begin soon after birth and last for 3 months for scotopic spectral sensitivity and 6 months for photopic spectral sensitivity. The critical period for visual acuity loss is much longer, lasting over 24 months. Binocular vision development can be disrupted by monocular deprivation starting as late as 25 months of age (roughly equivalent to 8 years in humans). However, the “end” of the critical period for binocular functions has not been determined for monkeys.
Because the sensitivity of the visual cortex to deprivations varies substantially during the critical periods, the timing of deprivation, i.e. onset and duration , has significant effects on the severity of perceptual and neural deficits. At what age is monocular form deprivation likely to have the most damaging effects in monkeys? The perceptual development of contrast sensitivity and visual acuity in monkeys is most vulnerable to monocular form deprivation during the first 5 postnatal months. A sharp drop of sensitivity to deprivation occurs after this initial period of heightened sensitivity, followed by a gradual decline over an extended period of time, i.e. > 24 months ( Fig. 40.6 ). ,
For ocular dominance plasticity in V1 of monkeys, the most severe shrinkage of ocular dominance columns for the deprived eye occurs with the earliest onset age, e.g. 1 week of age. The degree of the shrinkage becomes progressively smaller as the onset of deprivation is delayed and there is no obvious shrinkage if the onset is set at the 12th postnatal week. Thus, contrary to a classic observation, the ocular dominance columns in layer IVC of monkey V1 are most sensitive to monocular deprivation right after birth.
These behavioral and anatomical studies reinforce the clinical view that removal of dense congenital cataract combined with good optical quality lenses or correction of ptosis at the earliest possible postnatal time is essential to minimize the negative impact of monocular form deprivation in humans.
The critical period for ocular dominance in cats begins about 3–4 weeks of age when the optics of their eyes becomes relatively clear, peaks around 6–8 weeks, and gradually decreases during the next 12–14 weeks. A similar timing of the critical period for monocular deprivation has been reported for rats and ferrets with minor variations. The critical period is longer for monkeys than that in lower species and appears to be generally correlated with animal’s life expectancy. , It is difficult to determine the precise critical period of vision development for humans in part because of the difficulties associated with conducting experiments on human infants and dependence on clinical observations for data collection. Although the critical period for humans varies considerably for specific visual tasks as evidenced in animal studies, the critical period for experience-dependent changes in humans is thought to begin soon after birth (within 6 months or earlier), peak around 1–3 years of age, and decline slowly until 8–10 years of age or later.
Molecular mechanisms of ocular dominance plasticity
The molecular mechanisms of experience-dependent ocular dominance plasticity have been extensively studied in rodents because the visual cortex of rodents is in general organized similarly to that in higher mammals , and a wide range of genetic manipulations are readily accessible in rodents. Synaptic events following monocular deprivation ( binocular competition ) consist of an initial reversible reduction in functional connections to the deprived eye and rewiring of the upper layer long-range connections. , These are followed by extended periods of strengthening of the responses to the non-deprived eye and an eventual structural reorganization ( consolidation ) ( Fig. 40.7A ). The initial rapid changes occur as a result of imbalance in the strength of the input signals between the deprived and the non-deprived eyes. This imbalance disrupts the timing of the firing of action potentials between the pre-synaptic (LGN) and post-synaptic (V1) neurons for the deprived eye. Uncorrelated firing of action potentials between the pre-synaptic and post-synaptic neurons in V1 leads to a weakening of synaptic connections for the deprived eye while well-timed firing between the pre- and post-synaptic cells strengthens the synapses for the non-deprived eye (“ Hebbian synaptic plasticity ”). The timing of pre-synaptic and post-synaptic neuronal spiking appears to be also modulated by inhibitory neurons in the circuitry. These activity-dependent changes in synaptic strength also involve long-term potentiation (LTP) of input signals from the non-deprived eye and long-term depression (LTD) of signals from the deprived eye.

Closely associated with LTP and LTD synaptic plasticity is the role of glutamate receptors in cortical neurons, in particular, n-methyl- d -aspartate (NMDA), α-amino-3-hydroxy-5-methyl-4-isoxazolepropionic acid (AMPA), and γ-aminobutyric-acid (GABA) receptors ( Fig. 40.7B ). Excitatory synaptic transmission is mediated by NMDA and AMPA receptors while inhibitory synaptic transmission is regulated by GABA-Aα receptors. Each of these glutamate receptors has a broad range of critical roles in regulating the balance between excitation and inhibition and ocular dominance plasticity. ,
NMDA receptors are made up of three types of subunits (NR1, NR2A and NR2B). The normal postnatal changes in the expression of NR1 and NR2A or NR2B subunits, e.g. the NR2A/NR2B ratio is low at birth and gradually increases during postnatal development, are also experience-dependent. As a result, the developmental changes of the NMDA subunit expression due to monocular deprivation, i.e. the activity-dependent regulation of the NR2A/NR2B ratios, are intimately involved in regulating the plasticity of the visual cortex. For example, an increase in the NR2A or NR2A/2B ratios leads to the induction of LTP and to a heightened sensitivity of synapses to modification.
AMPA receptors, composed of GluR2 and either GluR1 or GluR3 subunits, are also involved in synaptic plasticity. Synaptic strength is determined by the AMPA receptor density and calcium permeability. Repetitive activation of synapses leads to increased insertion of AMPA subunits into postsynaptic neuronal membrane resulting in LTP whereas reductions in synaptic activation, e.g. by monocular deprivation, remove AMPA receptors leading to LTD.
Synaptic depolarization via activation of NMDA and AMPA receptors induces calcium influx and activates an intracellular signaling cascade . The second messenger molecules that are directly involved in synaptic strength and ocular dominance plasticity include protein kinase (PKA), calcium/calmodulin-dependent protein kinase II (CaMKII), extracellular signal-regulated kinase 1,2 (ERK), cyclic AMP responsive element binding protein (CREB), and protein synthesis machinery. PKA, CaMKII, and ERK rapidly promote ocular dominance plasticity by modulating synaptic strength by phosphorylating plasticity-regulating molecules in glutamate or GABA receptors. This kinase signaling leads to the activation of CREB. The changes initiated by activation of intracellular second messenger molecules along with the action of brain-derived neurotrophic factor (BDNF) leads to the enhanced expression of molecules that act on tissue plasminogen activation (tPA). BDNF and tPA can initiate the changes of dendritic spine motility, spine density, and extracellular matrix that ultimately result in rewiring of cortical circuits favoring the non-deprived eye.
GABA-mediated inhibition is important in maintaining the balance between cortical excitation and inhibition, hence in regulating the timing of ocular dominance plasticity. Manipulation of the normal level of GABAergic transmission in the developing brain can delay or advance the onset of the critical period. Preventing the maturation of GABA mediated transmission or dark rearing delays the critical period. Enhancing GABA transmission by infusing GABA agonist, e.g. benzodiazepines, or facilitating the growth of GABAergic interneurons by BDNF can advance the onset of the critical period. ,
The clinical significance of these manipulations to “reset” the excitatory and inhibitory balance in V1 by various pharmacological methods is that the results may provide new insights into the mechanisms underlying critical periods, and thus, reveal potential means to promote functional recovery in adults with developmental vision disorders. ,
Another class of molecules involved in synaptic plasticity is the neuromodulators including norepinephrine (noradrenaline), serotonin, and acetylcholine. These molecules are abundantly present throughout the cortex. Enhancing adrenergic, serotoninergic or cholinergic modulator systems facilitates the onset of ocular dominance plasticity. Changes in the expression of neuromodulators influence the level of LTP and LTD induction by modifying the intracellular calcium concentration by second messenger pathways and results in the associated structural reorganizations of local connections to the visual cortex.
Effects of early monocular defocus
Constant monocular defocus
A less severe form of monocular image degradation results from large differences in refractive errors between the two eyes ( anisometropia ). Normal primates begin their life with modest but binocularly balanced hyperopic refractive errors that decline to a normal refractive state during early infancy ( emmetropization ). , If infants have large differences in refractive state between the two eyes, they are unable to focus with both eyes. To avoid experiencing binocularly discordant images, infants begin to fixate with one eye (typically with the eye having a less severe refractive error) and as a result, the non-fixating eye experiences a defocused image. Monkey models of anisometropia are simulated by rearing infant monkeys with monocular defocusing lenses or by monocular atropinization.
Perceptual deficits
The perceptual consequences of untreated early anisometropia are generally similar to the anomalies found after early monocular form deprivation; impoverished binocular vision, reduced contrast sensitivity for high-spatial-frequency stimuli, and lower optotype acuity in the affected eye ( anisometropic amblyopia ). However, the perceptual deficits in anisometropes are generally less severe than in monocular form deprivation and are spatial frequency dependent ( Fig. 40.8A ). Anomalies vary substantially between individuals depending on the etiological factors and rearing histories, e.g. the degree of defocus.

Neural changes
Abnormal alterations in cortical physiology that result from early unilateral defocus are also similar to, but milder than cortical deficits in monocularly form-deprived monkeys. The ocular dominance distribution of V1 neurons is marked by a substantial loss of binocularly balanced cells (ocular dominance between 3 and 5) and by a milder but significant shift away from the affected eye ( Fig. 40.8B ). The sensitivity of V1 neurons to binocular disparity is substantially reduced and complex cells are more severely affected than simple cells ( Fig. 40.8C ). The spatial resolution and contrast sensitivity of V1 neurons for the affected eye of a severely anisometropic monkey are slightly lower than that of the fellow eye. , However, the magnitude of deficits in the spatial receptive-field properties of V1 neurons is too small to account for their perceptual loss in acuity and contrast sensitivity. ,
Alternating defocus
Early monocular defocus can be alternated daily between the two eyes to prevent the development of amblyopia. , If infant monkeys experience alternating defocus, the monocular response properties of V1 neurons, such as orientation selectivity, spatial frequency tuning, and/or spatial resolution, do not show response alterations that favor one eye over the other because each eye receives uninterrupted vision on alternate days during early infancy. However, daily alternating defocus leads to a spatial-frequency-dependent loss of local stereopsis (elevated disparity threshold) ( Fig 40.9B ). This reduction of local stereopsis is exaggerated for high-spatial-frequency stimuli because larger defocus generates greater conflicts between signals coming from the two eyes. Moreover, the disparity sensitivities of V1 neurons are significantly reduced in these monkeys and this reduction is also spatial frequency dependent.

These observations support the traditional view that local stereopsis is spatial frequency dependent, and that binocular disparity information is processed by independent channels tuned to different spatial frequencies. , It is also evident that the local disparity processing mechanisms in V1 can be independently compromised by early abnormal visual experience depending on their spatial frequency tuning properties. Finally, the effects of early alternating defocus on binocular vision development underscore the importance of having the normal presence of disparity-sensitive neurons in V1 for local stereopsis although disparity-sensitive V1 neurons alone are not sufficient to support fine stereopsis, i.e. it requires further processing by extrastriate neurons. ,
Effects of early strabismus
Strabismus is a chronic deviation of the visual axes that emerges shortly after birth. , The direction of the axis deviation can be convergent (esotropia), divergent (exotropia), or vertical (hypertropia). The etiology of infantile or congenital strabismus is not known. There is a clear familial tendency of developing strabismus, but the genetic factors responsible for infantile strabismus are not well understood. In primates, a high degree of uncorrected hypermetropia soon after birth is known to result in esotropia (accommodative esotropia).
Perceptual deficits
Strabismic infants experience double vision (diplopia) immediately after the onset of misalignment. If “normal” alignment is not achieved in a timely manner, binocular vision anomalies, such as deficient stereoscopic vision ( Fig. 40.10 ), reduced binocular summation of contrast sensitivity, and clinical suppression are likely to develop. Amblyopia may develop if strabismus is not treated for an extended period of time during the critical period. The effects of experiencing early strabismus have been extensively studied in monkeys by artificially creating ocular misalignment shortly after birth.
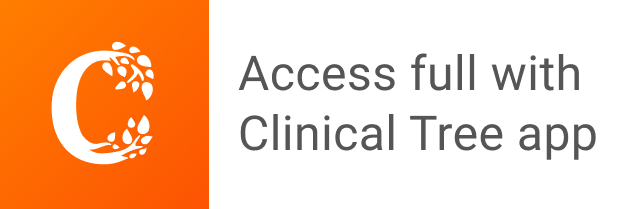