Abstract
Vestibular function is present at birth and is gradually modulated by developing central inhibitory influences, cerebellar control, visual development, and central vestibular adaptation until it reaches maturity at approximately 15 years of age. Children do not commonly complain of dizziness or vestibular dysfunction; therefore, the diagnosis relies on careful questioning of the child (if applicable) and parents, targeted imaging/testing, and an astute clinician to synthesize findings into a cohesive diagnosis. Abnormal responses in children require clarification to distinguish whether the locus of the problem rests primarily with the vestibular system, the vestibular pathways, or with abnormalities in visual, motor, or proprioceptive systems that jointly contribute to the acquisition of motor milestones. With a careful history and appropriate vestibular and balance testing, it is frequently possible to identify the likely cause of the balance disorder, even in the most complex patients.
Keywords
Differential diagnosis, Motor development, Vestibular maturation, Vestibulocollic reflex, Vestibulo-ocular reflex, Vestibulospinal reflex
Clinicians are just beginning to adequately recognize pediatric vestibular disorders as an area of significant importance in the overall well-being of children. Mandates for universal newborn hearing screening have resulted in an early focus on auditory function in infants, facilitating the early identification and management of children with auditory pathology. This has vastly improved outcomes for children with hearing loss and has resulted in a welcomed spillover of increased awareness that auditory and vestibular pathology frequently co-occur.
From the day we are born until we reach old age, we are profoundly dependent on our sense of balance for our well-being and survival. Balance competence relies on complex interactions and central mediation of three important sensory systems: vision, vestibular function, and proprioception. Structurally, sensory systems related to balance are fully developed at birth. From infancy, balance function continues to mature with a sequential acquisition of motor milestones, including head control, sitting, standing, and walking, and develops thereafter through experiential learning and adaptation until adolescence. Changes in balance function are most rapid and pronounced during infancy and in preschool years when motor milestones needed for walking are realized, and as postural control and coordinated movements are refined. Nevertheless, changes in balance function continue to be evident as a function of aging throughout the human lifespan.
Overview of the Vestibular System
The vestibular system is comprised of two otolith organs (the saccule and utricle), which sense linear acceleration (i.e., gravity and translational movements), and three semicircular canals, orthogonal with respect to each other, which are responsive to angular acceleration. The sensory hair cells of the otolith organs are located in the maculae and ampullae of the semicircular canals. Hair cell activation resulting from endolymphatic fluid flow (from head or body movement relative to gravity or a stationary position) generates afferent impulses that are transmitted to bipolar cells of the vestibular (Scarpa) ganglion, which houses cell bodies of vestibular afferent neurons. The axons of bipolar cells pass through the internal auditory canal (IAC) as separate superior and inferior divisions that come together as a single vestibular nerve. The superior vestibular nerve innervates the superior and lateral semicircular canals as well as the utricle. The inferior vestibular nerve innervates the posterior semicircular canal and the saccule. The vestibular nerve joins the cochlear nerve at the porus acusticus of the IAC, and together they travel through the cerebellopontine angle to the brainstem. These first-order neurons terminate in vestibular nuclei in the floor of the fourth ventricle without crossing the midline. The four major vestibular nuclei include the superior, lateral, medial, and descending vestibular nuclei. From the vestibular nuclei, axons project to the cerebellum, extraocular motor nuclei, antigravity muscles, and contralateral vestibular nuclei. Cortical representation of the vestibular system is at the level of parietal and insular regions of the cortex.
Embryology
Inner Ear
Ongoing research and technological advances have significantly improved our understanding of the cellular differentiation and morphogenesis of the human vestibular labyrinth. The first stages of inner ear development begin as diffuse thickenings of surface ectoderm on either side of the embryonic (rhombencephalon) hindbrain. During the third week of embryonic development, the thickened surface ectoderm from either side of the embryonic hindbrain begins to invaginate, forming the otic placodes.
During week 4, the otic placodes are surrounded by proliferating embryonic mesoderm, creating otic pits. The otic pits subsequently pinch off from the surface ectoderm to form the closed, rounded structures of the otic vesicles. The otic vesicles further differentiate into upper and lower portions, forming the membranous cochlea and the vestibular apparatus. Different rates of growth among the semicircular canals, vestibular aqueduct, oval window, round window, and cochlea have been observed, suggesting that each part of the inner ear follows distinct trajectories during development. The vestibular apparatus, located superiorly in relation to the cochlea, develops earlier and grows at a faster rate than the cochlea. The otic vesicle elongates and differentiates to form a dorsal utricular portion and a ventral saccular portion. The utricular portion becomes the semicircular canals and the utricle. Superior semicircular canals form first, followed by posterior and then lateral canals. The saccular portion becomes the saccule and the cochlear duct. The communication between the saccule and membranous cochlea narrows to form the ductus reuniens.
The bone that encapsulates the membranous labyrinth forms rapidly from the embryonic mesoderm over a period of approximately 5 weeks, between gestational weeks 19 and 23. Ossification of the otic capsule and encapsulation first occur in the area of the cochlea and superior semicircular canal at approximately 19 weeks’ gestation. Encapsulation then appears to progress in an outward fashion from areas surrounding the vestibule to the canal vertices, with the last area of encapsulation being the posterolateral area of the horizontal semicircular canal at approximately 21–23 weeks’ gestation. Current consensus is that the vestibule is adultlike in form and size by 25 weeks of gestation; however, recent findings suggest that some parts of the labyrinth only reach the final size after birth. This seems to be the case for the internal aperture of the vestibular aqueduct, which is still growing and smaller than adult size at 39 weeks’ gestation.
Maturation of Vestibular Receptors
Around the third week of gestation, sensory epithelia develop from the ectoderm in the cristae of the forming semicircular canals and in the maculae of the forming otolith organs. By 7 weeks of gestation, small quantities of otoconia are present in the utricle. Thereafter, development proceeds quite rapidly, and within 1 week more otoconia are present in both the utricle and saccule, and cellular differentiation of the macular neural substrate is readily evident. Observations during the period of 7–12 weeks’ gestation show that the calcium content of otoconia increases markedly in both the utricle and saccule; however, comparisons along the entire continuum of macular development reveal that the otoconia of the utricle appear to be more mature and varied in size and shape than saccular otoconia. Vestibular hair cells first appear at approximately 7 weeks’ gestation. Although vestibular hair cells are not fully differentiated, the beginnings of synapse formation in vestibular hair cells are observed in the human fetus at approximately 9–10 weeks’ gestation. Differentiation of type I and type II hair cells begins between 11 and 13 weeks’ gestation. In general, the morphologic sequence is from apex to base in the cristae and from center to periphery in the maculae. Significant numbers of fully formed calyx nerve endings are observed at 20 weeks’ gestation. The maturing ampullary cristae become active as early as the eighth or ninth week of fetal life. Reflexogenically, vestibular receptors become fully active by the 32nd week of gestation, at which time a fully developed Moro reflex can be elicited. These observations suggest that vestibular afferents are mature and functional in the early stages of human development.
Development of vestibular pathways
Vestibular ganglion cells are of various shapes until the 21st week of gestation and become uniform in shape around the 24th week of gestation when development of the inner ear is complete. Morphometric studies show that ganglion cells grow until the 39th week, reaching maturity around the time of birth. Neuronal connections between the labyrinths and the oculomotor nuclei in the brainstem occur between the 12th and 24th weeks of gestation. Myelination of the vestibular nerve begins around the 20th fetal week; it is the first cranial nerve to complete myelination. The vestibular nuclear complex is functional at 21 weeks’ gestation.
Developmental Reflexes
With the maturation of physiologic processes and anatomical structures, certain developmental reflexes can be elicited at birth or soon thereafter. These are primitive in nature, usually disappear as the child matures, and primarily reflect integrity of the brainstem and spinal cord. Their persistence beyond the normally expected age of dissipation indicates delayed maturation or impaired nervous system function. Asymmetry of these reflexes suggests either a central or a peripheral nervous system disorder.
The Moro reflex is elicited by holding the child supine and allowing the head to drop approximately 30 degrees in relation to the trunk. Extension and abduction of the arms with fanning out of the fingers, followed by adduction of the arms at the shoulder, takes place as a normal response. This reflex normally disappears by age 5–6 months.
The tonic neck reflex is tested by turning the head of the child to one side while the child is lying supine with shoulders fixed. The arm and leg toward the side that the head is turned extend, whereas the arm and leg on the opposite side flex. This reflex normally disappears by the age of 6 months.
The head righting reflex develops by the age of 4–6 months. To test this reflex, the child’s trunk is held 30 degrees from the vertical. A normally responding infant will then tilt the head so that it remains vertical. At approximately 5 months, the child will additionally move the lower limbs away from the side to which they have been tilted, thereby signaling functional integration of visual, vestibular, and proprioceptive stimuli.
The parachute reaction can be elicited in infants over the age of 5 months with a sudden downward movement of a vertically held child, which causes the lower limbs to extend and abduct. This reflex is considered to represent visual-vestibular interaction with the otolith organs presumably involved.
The Doll’s eye response is found normally in full-term babies within 2 weeks of birth. When the baby (facing the examiner) is held at arm’s length and rotated in one direction around the examiner, a deviation of the eyes and head opposite to the direction of the rotation is produced, representing vestibular activity. Owing to an immature saccadic system at this stage, the fast component of a normal nystagmic response is not seen. Later, however, nystagmus is apparent, with the fast component in the direction of rotation.
Development of Vestibular-Induced Reflexes
Balance and equilibrium are maintained through a series of events triggered by sensory stimulation. Incoming sensory inputs received from vestibular, visual and somatosensory/proprioceptive systems are directed to the vestibular nuclei and cerebellum for processing and calibration. In response to afferent inputs, the vestibular nuclear complex creates direct and remarkably rapid efferent connections to muscles controlling eye movement, as well as the neck and spinal cord. These motor outputs stimulate three vestibular reflexes (vestibulo-ocular vestibulospinal, and vestibulocollic), which allow us to maintain control of our gaze, postural stability, and our balance and equilibrium. It is through examination of these reflexes that we are provided a window for uncovering vestibular dysfunction. Understanding how vestibular responses differ among infants, children, adolescents, and adults is crucial when attempting to evaluate and diagnose vestibular pathology.
Vestibulo-ocular reflex
The purpose of the vestibulo-ocular reflex (VOR) is to stabilize gaze and maintain clear vision when the body or head is in motion. Objects of visual interest are maintained on the fovea of the retina through inputs from semicircular canals and otolith organs.
Historically, data regarding VOR function in infants and children have been somewhat limited owing to technical difficulties inherent in achieving compliance and obtaining accurate recordings. The VOR is also subject to alteration from a variety of nonvestibular influences, including subject attention and state of arousal, unintended ocular fixation due to light leaks, inadequate calibration, and insufficient head stabilization during testing. In several decades of research with children, many techniques have been used to explore and record the pediatric VOR. These include caloric stimulation, rotational (torsion swing) stimulation, and passive whole body (en bloc) rotation techniques. Depending on the technique used, parameters such as the speed of the slow component of eye movement (expressed in degrees per second), amplitude of nystagmus beat, and latency and duration of response have been recorded. Researchers using en bloc rotational techniques have explored factors such as gain (the ratio of peak eye velocity to peak head velocity), phase (the timing difference between head and eye velocities), symmetry (the comparison of rightward and leftward eye velocities), and vestibular time constant (the number of seconds required for the slow-phase eye velocity to decline by two-thirds of its maximum value).
The VOR is present at birth; however, vestibular time constants are approximately one-half of normal adult values in neonates aged 24–120 hours. Vestibular time constants appear to approach adult values by 2 months of age. These differences are a reflection of the immaturity of visual pathways at birth, which indicates that maturation of visual pathways is a necessary precursor for adequate calibration of the VOR and for competent function of velocity storage mechanisms necessary for stable vision. Reflexive slow component nystagmus of the VOR generated by vestibular stimulation is routinely observed at birth; however, the centrally mediated fast component, which returns and maintains the eyes within the physical confines of the orbits, is variably present. Infants demonstrate inaccurate saccades, frequently requiring more than one saccade to reach the target. The saccadic system is immature at birth, continuing to develop up to the age of 2 years. The velocity of the VOR slow component as well as the frequency of nystagmic beats increase as a function of age until age 6–12 months, after which values reach a plateau and stabilize. Smooth pursuit is also only possible at very low frequencies in neonates because of foveal immaturity. A higher gain of the VOR in response to sinusoidal rotation is observed in children compared with adults and is due to immature visual-vestibular interaction, with poorer suppression of the VOR response. VOR gain and vestibular time constants in response to sustained angular acceleration reveal that vestibular time constants increase, whereas VOR gain shows small but significant decreases as a function of age from 2 months to 11 years. In a recent large longitudinal study, Casselbrant et al. observed that, in response to both sinusoidal and constant velocity rotations on an earth vertical axis, VOR gain increases linearly (ratio of peak eye velocity to peak head velocity) as a function of age from 3 to 9 years but phase differences appear to remain stable. These findings are in contrast to several other studies that have shown a decreased or stable VOR gain as a function of a child’s increasing age.
In summary, the human VOR goes through several developmental stages, with a healthy response developing by several months beyond full term, and absence of the VOR by the age of 10 months should be considered an abnormal finding. It is evident that regardless of the parameter explored, the underlying prevailing motif across all studies of pediatric VOR is that qualitative differences exist between the VOR function of children and adults and that these differences seem to persist until preadolescence.
Vestibulospinal reflex
Whether the body is stationary or in motion, continuous afferent signals from vision and vestibular inputs detect the body’s orientation and relationship to gravity. These inputs combine with touch receptors of the skin as well as proprioceptors on the soles of the feet, the hands, and torso to detect the body’s contact with the environment. The sum of these inputs provides the information needed to generate the vestibulospinal reflex (VSR), which stabilizes the body and maintains postural control. VSR signals travel along three major pathways, including the lateral, medial, and reticulospinal tracts. When activated, these neural tracts impact anterior horn cells of the spinal cord and generate myotactic, deep tendon reflexes in antigravity skeletal muscles of the limbs and trunk.
The VSR has more numerous and complex innervations than the VOR, but just as the VOR works to contract and relax paired ocular muscles, the VSR works to create a push-pull arrangement of agonist and antagonist muscle groups firing across the neural axis. A variety of diagnostic tests exploring aspects of VSR function have been developed for use with both children and adults. In general, when comparing the VSR function of children with that of adults, as noted by Rine, the postural control of these groups varies significantly. As detailed later in this chapter, the vestibulospinal mechanism and effectiveness of the vestibular system in postural control continues to develop until at least 15 years of age.
Vestibulocollic reflex
The vestibulocollic reflex (VCR) plays an important role in stabilizing vision by compensating for head movements when the body is in motion. Through patterned contractions of neck muscles, the VCR stabilizes the bobbing of the head caused by vibrations transmitted from the heels as they strike the ground during walking and running. Thus the VCR assists in stabilizing the head on the neck and in keeping the head still and level, especially during ambulation. As we walk, vestibular signals caused by linear translations stimulate nerve receptors of the saccule. In response, the saccule transmits afferent signals along the inferior vestibular nerve and ganglion to the vestibular nuclear complex in the brainstem. From vestibular nuclei, efferent signals are sent via the medial vestibulospinal tract and spinal accessory nerve to neck muscles, including the sternocleidomastoid muscle, one of the long neck muscles extending from the thorax to the base of the skull behind the ear.
In the last decade, VCR function has become routinely explored through recordings of vestibular evoked myogenic potentials (VEMPs). VEMPs have become an increasingly popular clinical technique because, unlike other tests of vestibular function, VEMPs provide information regarding saccular and inferior vestibular nerve function. This is a significant benefit because otolith organs and anterior and posterior semicircular canals may be more instrumental in locomotion and posture control than horizontal semicircular canals explored by the VOR. In addition, the VEMP test is an objective measure that can be reliably recorded from surface electrodes in a wide variety of patients, including infants and young children. VEMPs are stimulated by high-intensity auditory stimuli that cause robust vibration of the ossicular chain and stimulate the saccule, which rests in close proximity. Neural impulses traveling along the VEMP neural pathway stimulate the VCR, creating an efferent inhibitory release of the tonically contracted sternocleidomastoid muscle. VEMP recordings appear as a biphasic EMG potential, with an initial positive deflection at 13 ms post stimulus onset (P13) and a negative deflection at 23 ms (N23). A word of caution is required regarding high stimulus intensities necessary to elicit VEMPs. When a stimulus is delivered to the small ear canal of a pediatric patient, stimulus intensity can reach 130–135 dB, which can be painful and potentially damaging to hearing.
Studies recording VEMPs in preterm neonates, infants, and young children have confirmed the efficacy of VEMP recordings in the pediatric population. These studies have pointed out differences between VEMP responses of children and adults, suggesting again maturational effects from preschool age through adolescence.
Balance and Motor Development
Maintenance of postural balance requires an active sensorimotor control system. In adults, the sensory systems are well organized and act in a context-specific way. Postural control involves sensory feedback, and visual and proprioceptive inputs need to be integrated in order for the center of foot pressure to move in phase with the body’s center of mass. In children, the sensory systems are not completely developed, although their anatomic structures are mature early in life. Proprioceptive, visual, and vestibular systems develop more slowly than the hierarchically lower automatic motor processes that mature early in childhood. The importance of visual cues in maintaining static posture has been well demonstrated, particularly in children who are accustomed to visually monitoring the body during postural adjustments. As important are cognitive functions for organization and integration of available sensory information under both static and dynamic conditions, and this has also been well documented. Hence, selection of the appropriate balance strategy not only depends on environmental demands but also is a function of neuromaturation and experience.
In typically developing children, postural stability proceeds in a cephalocaudal fashion, with the infant first achieving control of the head, then the trunk, and finally postural stability in standing. The newborn infant, when held ventrally with a hand under the abdomen, cannot hold up the head. By 6 weeks of age the head is held in the plane of the body and by 12 weeks, above this level. Head control, allowing the baby to look around in a horizontal plane, is achieved by 16 weeks of age, and by the 36th week, the infant is able to sit unsupported for a few minutes. By the age of 1 year, the child is able to crawl on hands and knees and stand up holding on to furniture. At approximately 15–16 months of age the child is normally able to start walking.
Coordination of postural responses develops until at least 10 to 15 years of age. In balance control, somatosensory inputs receive priority in adults, whereas children prefer visual inputs to vestibular information in achieving their postural equilibrium. Infants and young children (aged 4 months to 2 years) are dependent on the visual system to maintain balance. At 3–6 years of age, children begin to use somatosensory information appropriately, although some studies indicate that development continues until the age of 9–11 years. In the case of an intersensory conflict, the vestibular system provides a referential function by suppressing any input that is not congruent with vestibular information. Owing to presumably mature vestibular function, even with misleading visual information, adults are able improve their postural control. Children by age 12 years are still not able to select and process misleading visual information. Among the three sensory inputs in children, the vestibular system seems to be the least effective in postural control, and functional efficiency of the vestibular system in children of 10–15 years of age is still developing. Development of several visual functions, such as saccade latency, contrast sensitivity, or chromatic sensitivity, does not approach adult levels until approximately 12 years of age. The visual influence on standing stability is reported to be established at adult levels around the age of 15 years. Adult-like postural stability resulting from complete maturation of the three integral sensory systems and the ability to solve intersensory conflict situations can thus be assumed in adolescents around 15 years of age.
In summary, vestibular function is present at birth but continues to mature so that it is most responsive between 6 and 12 months of age. Subsequently, vestibular responses are gradually modulated and refined by developing central inhibitory influences, cerebellar control, visual development, and central vestibular adaptation and reach adultlike maturity around 15 years of age.
Incidence of Vestibular Disorders in the Pediatric Population
The general prevalence of pediatric vestibular dysfunction is estimated between 8% and 18%, although the incidence of vertigo as a primary complaint in a review of hospital records was less than 1%.
The Differential Diagnosis
Children do not complain about vestibular dysfunction, and therefore the diagnosis relies on careful questioning of the child (if applicable) and parents, targeted imaging/testing, and an astute clinician to synthesize the findings into a cohesive diagnosis. Abnormal responses in children need further clarification to distinguish whether the problem rests primarily with the vestibular system and vestibular pathways or with abnormalities in visual, motor, or proprioceptive systems, which jointly contribute to acquisition of motor milestones.
Patient History
Vertigo, defined as the subjective sensation of movement, can be difficult for many patients to describe. This is intrinsically the case because central vestibular projections to the cerebral cortex are diffuse. This challenge is even more apparent in the pediatric population where children do not have the breadth of experience or vocabulary to describe this sensation. As such, vestibular disorders in children can be difficult to recognize. Children with vestibular disorders are often written off as clumsy or uncoordinated, or dysfunction is misjudged to be secondary to a behavioral abnormality. Children may present with complaints of abdominal pain, ataxia, headache, visual disturbance, hearing loss, otalgia, or otorrhea. On the other hand, children with vestibular disorders may have no complaints. In a study of 62 children with basilar skull fracture, 34% of whom had sensorineural hearing loss (SNHL), “few” of the children had vestibular complaints.
It is frequently possible with a careful history, engaging both the child and caregiver, to identify the likely cause of the balance disorder even in the most complex patients. A series of focused questions, based on the excellent work by G. Michael Halmagyi, helps differentiate the nature of the pathology in most patients ( Table 3.1 ).
Question | Clinical Information |
---|---|
What does it feel like? |
|
What other symptoms are associated with it? |
|
How long do the symptoms last and how many have occurred? |
|
What makes it better or worse? |
|
What is the background history? |
|
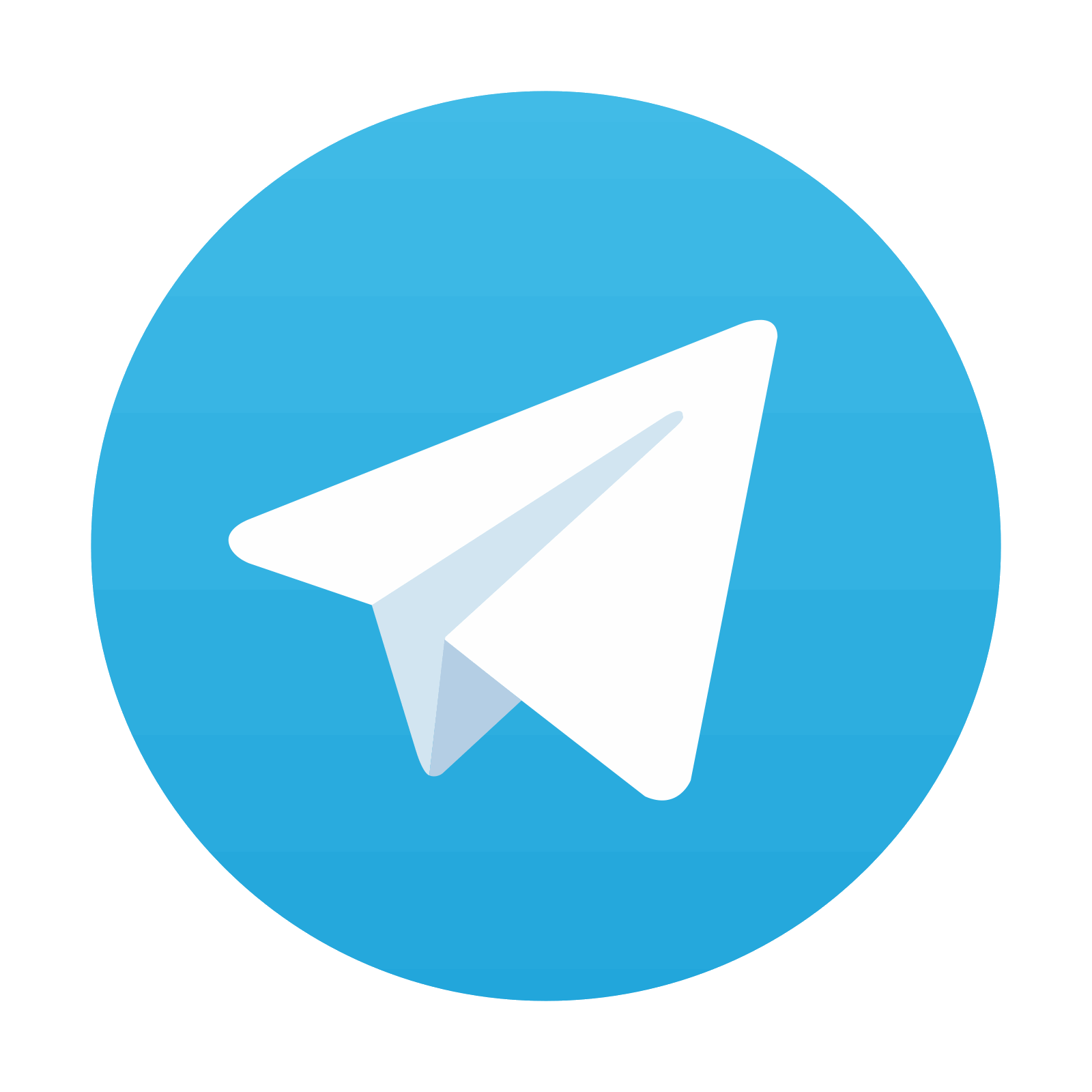
Stay updated, free articles. Join our Telegram channel
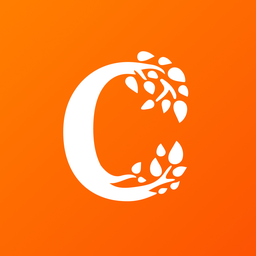
Full access? Get Clinical Tree
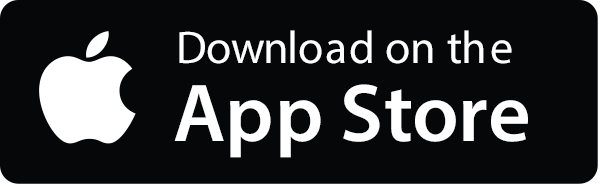
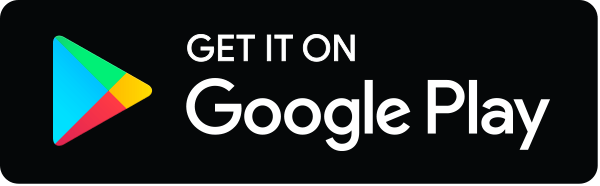
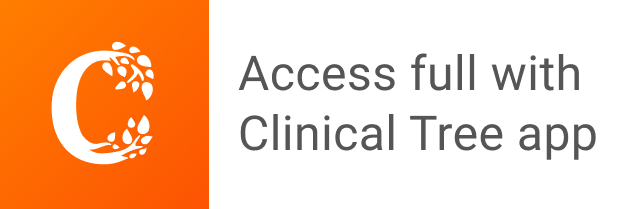