Introduction
The dorsal lateral geniculate nucleus of the thalamus (LGN) is the gateway through which visual information is transmitted from the retina to the cortex; therefore, conscious visual perception relies on the ability of LGN neurons to faithfully relay the specific features of the visual world that are encoded by the retina. Accordingly, the connections between retinal ganglion cells (RGCs) from each eye and LGN neurons are highly precise and organized. A key feature of retinogeniculate organization that allows for binocular vision is that axons arising from each eye are segregated within the LGN. Additionally, RGC projections are arranged such that neighboring cells in the retina innervate neighboring cells in the LGN, creating a retinotopic map in the thalamus that is capable of maintaining the spatial features of the visual scene. The resolution of the retinal map is also maintained in the LGN; consequently, LGN receptive fields are similar to those of RGCs in their size and structure. Finally, some cell type–specific organization also exists in the LGN, since different types of LGN neurons reside in distinct laminae and receive inputs from different subtypes of functionally distinct RGCs.
How is such precise retinogeniculate circuitry established during development? First, RGC organization must be determined and maintained during the path from eye to brain. Numerous studies have demonstrated that upon RGC growth into the LGN, many aspects of retinogeniculate connectivity are initially imprecise, and that the immature circuit must subsequently undergo refinement to achieve its finely tuned mature form. Much of this refinement occurs before the onset of vision. It is now well established that this early refinement requires spontaneous retinal activity and molecular cues that are present throughout development, and that the non-neuronal cells of the brain, glia, are important players in sculpting the mature circuitry.
Formation of eye-specific territories
Before reaching their eventual brain targets, RGCs exit the retina as a fasciculated bundle via the optic stalk, which then becomes the optic nerve. At the optic chiasm, the crucial choice point in RGC axon path determination, RGCs partially decussate: some RGC axons cross to the opposite-side hemisphere of the brain (contralateral RGCs) and other RGC axons remain on the same-side hemisphere of the brain (ipsilateral RGCs). Following partial decussation at the optic chiasm, when these axon populations are interleaved, RGCs regain topographic and eye-specific organization and refasciculate to form the optic tract, via which RGCs travel until they reach their final brain targets, including the LGN.
Decussation of ipsilateral and contralateral RGCs at the optic chiasm enables binocular vision by ensuring that each visual hemifield is adequately represented in both brain hemispheres. The extent of RGC decussation reflects evolution of eye placement—as organisms have evolved to have eyes more frontally positioned, the ratio of ipsilateral-to-contralateral axon projections has increased, with humans having a 45:55 ipsilateral-to-contralateral ratio, whereas animals with more laterally positioned eyes, such as fish, birds, and amphibians, have a much lower ipsilateral-to-contralateral ratio. In mice, the most utilized model system for studying visual pathway development, the ipsilateral-to-contralateral ratio is about 5:95.
The optic chiasm is a crucial choice point in establishing RGC axon projections
The decision to cross onto the opposite side of the brain or to stay on the same side of the brain is molecularly guided. Developing RGC axons in the optic nerve grow among interfascicular glia. At the optic chiasm midline, radial glia at the floor of the third ventricle form a palisade through which RGC axons extend. This glial palisade is instructive for RGC navigation, as it expresses ligands to receptors on RGC growth cones that direct growing RGC axons to either cross or avoid the midline.
The receptor-ligand pairings that determine RGC midline choice at the optic chiasm are specific to each of these RGC axon subtypes, and RGC axon subtype is, in turn, dependent on the expression of distinct subtype-specific transcription factors that direct receptor expression. Ipsilateral RGC fate is principally determined by the transcription factor Zic2. Zic2 drives the expression of the EphB1 receptor in ipsilateral RGCs, which interacts with radial glia–expressed EphrinB2 to drive RGC axon repulsion away from the midline and back into the ipsilateral optic tract.
Determination of contralateral RGC identity and midline crossing is comparatively more complex, with contralateral RGC fate specified by a consortium of transcription factors including Islet2, Brn3a, and SoxC. Midline glial and contralateral RGC axon signaling is similarly combinatorial, dependent on NrCAM, Sema6D, and Plexin-A1, VEGF-A/Neuropilin1 signaling, and noncanonical Wnt signaling, which functions dually in RGC sorting at the midline, playing a role in both ipsilateral midline repulsion and contralateral midline attraction.
Intriguingly, axon-axon interactions have also been shown to mediate axon choice at the optic chiasm: Sonic hedgehog (Shh) is produced by contralateral RGCs at the retina and transported anterogradely along the axon to the optic chiasm, where it accumulates and repels ipsilateral RGCs via Boc receptor signaling. Axon-axon interactions independent of midline crossing signals also mediate eye-specific axon organization in the optic tract, but the mechanisms guiding this interactions are still being elucidated.
Molecular mechanisms guiding the formation of eye-specific axonal territories
About 40% of all RGCs project to the LGN, where they are organized by eye specificity, topography, and subtype identity. Although recent advances in single-cell genomics have allowed for increasingly greater resolution into RGC molecular identity and the subsequent delineation of additional RGC subtypes, little is known about the mechanisms underlying molecular RGC subtype-specific patterning in the LGN. Indeed, eye-specific and topographic patterning in the LGN has been studied for far longer, although the mechanisms guiding RGC axon selection of appropriate eye-specific and topographic targets remain poorly understood. However, timing-based mechanisms and signaling via the Eph/Ephrins and homophilic Teneurin adhesion family members mediate retinotopic and eye-specific projections, respectively.
Retinogeniculate projections are refined during development
Anatomical studies have shown that when the axons from the two eyes initially invade the LGN, their arbors extensively overlap, and functional studies have demonstrated that these overlapping projections give rise to binocularly innervated LGN neurons. After this initial connectivity is established, there is a specific developmental window during which the axons from each eye segregate into nonoverlapping territories. This eye-specific segregation results in a pattern of ipsilateral and contralateral projections that is highly stereotyped both in size and placement within the LGN.
Following the formation of eye-specific territories, RGCs undergo further refinement. Whereas initially multiple retinogeniculate projections converge onto LGN target neurons, these projections have been understood canonically to refine to a near one-to-one ratio, with early studies showing that LGN neuron firing was nearly always preceded by the firing of a single, connected RGC. Such a one-to-one view of the mature retinogeniculate synapse has been challenged in recent years, with studies using modern anatomical and electrophysiological recording techniques revealing a higher degree of anatomical convergence onto LGN relay neurons than previously understood. These studies estimate an average of 10 to 14.5 RGCs per relay neuron in the mature LGN. Importantly, these inputs are heterogeneous in strength, with only a minority of RGC inputs strong enough to drive postsynaptic firing alone, and functional convergence is limited, with most adult LGN neurons responding to dominant input from one and not both eyes.
Activity-dependent refinement of retinogeniculate projections
Once it was appreciated that much of the initial development and refinement of retinogeniculate projections occurs before eye opening, these findings raised the question of whether synaptic transmission and/or neuronal activity are required for refinement ( Fig. 39.1 ). The first demonstrations that action potentials are required for eye-specific segregation came from studies in which tetrodotoxin (TTX) was used to block sodium channels in fetal cat brain. This activity blockade prevented the formation of eye-specific domains. Further experiments found that spontaneous activity is generated in the retina during eye-specific segregation, and when spontaneous retinal activity is disrupted, eye-specific layers do not form and receptive fields are larger than normal. Whereas the existence of spontaneous retinal waves was once controversial, the development of tools that enable these waves to be visualized and recorded in vivo have not only confirmed the existence of spontaneous waves in the retina but have also allowed for their characterization: waves propagate in a directionally biased manner, are weakly correlated between the two eyes, and produce corresponding waves of activity in postsynaptic neurons in the LGN, superior colliculus (SC), and visual cortex.

What parameters of activity drive refinement?
Three stages of patterned wave activity have been described in the developing retina. The activity at each stage is mediated by distinct mechanisms and displays unique properties, such as the frequency of the activity, the area of the retina involved in the correlated activity, and the speed of the propagating wave. These studies suggest that the unique features of these stages might contribute to the specific aspects of refinement that occur during each stage. The first stage of retinal activity, stage I, consists of infrequent bursts of patterned activity that appear embryonically and are largely mediated by communication through gap junctions. The bulk of retinogeniculate refinement occurs during stage II waves. Stage II waves are mediated by acetylcholine release from starburst amacrine cells and occur embryonically in mammals born with their eyes open and postnatally in mammals born with their eyes closed. The final stage of patterned spontaneous activity, stage III, is mediated by glutamatergic transmission from bipolar cells, spans the period of eye opening, and is propagated, at least in part, due to glutamate spillover.
Although most patterned spontaneous activity occur before eye opening, the developing retina responds to light passing through the closed eyelid. Intrinsically photosensitive RGCs (ipRGCs) emerge and respond to light during stage II cholinergic waves and rod- and cone-mediated light responses are observed in RGCs with the onset of stage III glutamatergic waves. Intriguingly, both stage II and stage III waves can be modulated by light, with effects on eye-specific segregation.
A common mechanism that appears to underlie the development of connection specificity throughout the nervous system is activity-dependent competition among presynaptic inputs for postsynaptic space. Evidence that eye-specific segregation involves competition among RGC inputs comes from studies in which activity was either blocked or enhanced in one eye, resulting in alterations to the pattern of ipsilateral and contralateral projections. In these studies when epibatidine was used to block cholinergic transmission in just one eye, the projections from the blocked eye lost territory in the LGN while the projections from the active eye expanded their coverage. The converse was also true; that is, when activity was increased in one eye by intraocular administration of either forskolin or the cyclic AMP analog, CPT-cAMP (which increase the size, frequency, and speed of waves), the projections from the more active eye gained territory in the LGN at the expense of the nonenhanced eye’s projections ( Fig. 39.2 ).

Both experimental and theoretical studies suggest that in addition to overall activity levels, specific aspects of patterned spontaneous activity may drive segregation through Hebbian mechanisms that strengthen and eliminate synaptic inputs over time. The Hebbian model of synaptic competition predicts that ganglion cells that “fire together, wire together.” For Hebbian mechanisms to drive segregation, neighboring RGCs must fire in a correlated manner, while more distant RGCs or RGCs from different eyes must exhibit less correlated spiking, thereby allowing for the strengthening of the temporally correlated neighboring inputs and the weakening of the uncorrelated inputs. Indeed, these requirements are met as a consequence of wave propagation. For example, during a wave, neighboring RGCs are coactivated, causing their firing to become more synchronized than that of non-neighboring RGCs.
Selectively altering the spatiotemporal properties (i.e., bilateral synchrony, frequency, and directionality ) of waves can result in both improper topographic map formation and eye-specific segregation. These studies indicate that certain characteristics of retinal waves are instructive for the proper development of RGC projections, with wave synchrony and frequency being particularly important for eye-specific segregation in the LGN.
Cellular and molecular mechanisms of RGC axon refinement
A major area of research is aimed at elucidating the molecular mechanisms that translate changes in synaptic drive into changes in synapse and axon morphology. Screening of transgenic mouse lines that display normal retinal waves yet have disrupted eye-specific segregation has demonstrated that several types of immune-related molecules are likely to be involved in this process. The first such example was the class I major histocompatibility complex (MHC). This protein is upregulated by activity in the LGN during the time of eye-specific segregation, and MHC I knockout mice display overlapping eye inputs. RGCs in transgenic mice lacking expression of neuronal pentraxins (NPs) and the classical complement cascade initiating protein C1q also fail to fully segregate into eye-specific territories.
Glia have emerged as major regulators of activity-dependent developmental refinement of RGC synapses. Transgenic mice independently lacking components of both microglial and astrocytic phagocytosis pathways show defects in eye-specific segregation. Manipulation of stage II spontaneous activity via the induction of competition between ipsilateral and contralateral eyes resulted in the preferential engulfment of weaker inputs by both microglia and astrocytes. Thus, both microglia and astrocytes contribute to eye-specific RGC segregation by actively engulfing weaker LGN synapses during early postnatal development.
Paralleling immune system phagocytes, microglia in the LGN appear to respond to “eat-me” or “don’t-eat-me” signals from RGC synapses via activation or inhibition of the classical complement cascade. Neuronal expression of C1q drives deposition of C3 (“eat-me”) onto RGC synapses, which tags these synapses for microglial engulfment via binding of microglial CR3 receptors. This process can be blocked by neuronal expression of sushi repeat protein X-linked 2 (SRPX2), which binds C1q directly and inhibits its activity. Conversely, microglial phagocytosis can be inhibited via neuronal expression of CD47 (“don’t-eat-me”), which binds microglial SIRPα. CD47 is preferentially expressed on more active RGC synapses, protecting these more active synapses from microglial phagocytosis.
The molecular mechanisms regulating activity-dependent astrocyte phagocytosis of RGC synapses during eye-specific segregation are not yet fully defined but involve cooperation of the MERTK and MEGF10 signaling pathways. Astrocyte phagocytosis of RGC synapses is also modulated by ApoE isoform in the LGN. The apparent redundance of microglial and astrocytic phagocytic pathways during activity-dependent eye-specific segregation underscores the importance of glial pruning, and whether or how these different pathways and cell types interact is an open area of investigation.
RGC synapse refinement is not exclusively the result of structural pruning within the proper eye-specific territory —rather, labeling and detailed reconstruction of individual RGC arbors from P8 to P31 showed that spatial redistribution of axon boutons underlies late synapse refinement and experience-dependent plasticity at the retinogeniculate synapse, with average arbor size and complexity actually increasing during this period of time. At P8, boutons are relatively evenly distributed across the RGC axon arbor. By P20, however, boutons have grouped into larger, dense clusters without restricted axon arbor regions, even while average number of boutons remains unchanged. In mice that are deprived of light exposure from P20 to P31, axon size and complexity are unchanged, but bouton clusters are smaller and more spaced out along the axon arbor when compared with those in age-matched mice raised in standard light conditions, indicating that sensory experience may drive small-scale changes in axon bouton rearrangement. This period of sensory experience–dependent synapse remodeling via bouton rearrangement is followed by a period of fine-scale structural pruning, with total axon length, complexity, and bouton density decreasing as remaining boutons grow in size from P31 to P60.
Microglia and immune molecules have also been shown to be involved in RGC refinement during sensory experience in a nonphagocytic manner. Concurrent with the latter phases of axon bouton rearrangement described previously, sensory experience–dependent retinogeniculate refinement is associated with the simultaneous strengthening of select synapses and elimination of weaker synapses. Mice lacking the cytokine receptor fibroblast growth factor–inducing protein (Fn14) have more, smaller synapses during this phase of refinement, indicating failure in both synapse strengthening and elimination. Visual experience also induces the transcription of Fn14’s ligand, TNF-associated weak induced of apoptosis (TWEAK), in a subset of microglia. Fn14 expression alone function to increase the number of bulbous spines on LGN relay neurons, strengthening synapses in response to visual experience. However, when Fn14-expressing retinogeniculate connections are near TWEAK-expressing microglia, TWEAK suppresses this Fn14-dependent increase in spine number. This represents a phagocytosis-independent mechanism by which microglia shape synapse refinement.
Beyond eye specificity
Recent advances in single-cell sequencing have led to the identification of at least 40 different functional RGC subtypes, with an estimated 75% of these subtypes projecting to the LGN. In addition to eye-specific segregation, RGC axons undergo further restriction of their arbors into functionally distinct sublaminae. For example, major RGC classes can be subdivided into two subtypes: cells that are depolarized (ON ganglion cells) or hyperpolarized (OFF ganglion cells) by light onset. At maturity these parallel ON and OFF pathways are segregated in the LGN at the level of single neurons; individual LGN neurons generally respond to increments or decrements in light but not both. In some species, such as the ferret, LGN neurons receiving ON input and OFF input reside in two distinct sublaminae within each eye-specific layer, and these sublaminae develop just after eye-specific segregation and before opening.
Other examples of functional classes of RGCs that project to the LGN are Y-cells, which process movement, X-cells, which are responsible for image acuity, and a heterogeneous population of W-cells. Whether individual LGN neurons initially receive synaptic input from these multiple classes of ganglion cells is unclear. In the primate, however, magnocellular and parvocellular pathways project to distinct regions of the LGN from early stages as their axons innervate targets. This suggests that during primate development single LGN neurons may receive inputs from just one class of RGC. This specificity could be due, at least in part, to the generation of different RGC classes at different times during development, and to the fact that the axons of parvo cells reach the LGN before those of magno cells. In addition, in primates the axons from the two eyes may initially innervate the LGN in a somewhat eye-specific manner; thus, the primate retinogeniculate connection may require less refinement than that of other mammals.
Interestingly, studies also suggest that once the mature pattern of anatomical and functional connections has been established, they must be actively maintained. Taken together, these data indicate that the mature retinogeniculate connection must be sculpted out of an initially overconnected circuit, and that the extent of this refinement may vary from species to species. These data also suggest that retinogeniculate development occurs over several stages beginning with the segregation of eye-specific inputs, followed by a more fine-scale refinement phase that sharpens the retinotopic map, and a final maintenance phase during which this connection remains malleable.
Summary
The mature retinogeniculate pathway is highly organized in structure and function, and a clearer understanding of how this precise organization develops continues to emerge. Organized RGC growth and targeting, as well as axon refinement, shape this final organization. Specific features of spontaneous retinal activity appear to be critical, suggesting that activity may work in an instructive fashion. An important task for future studies will be to further elucidate the cellular and molecular mechanisms, including participation by glial cells, that turn activity into structural changes in this pathway. The use of transgenic animals, additional genetic and molecular screens, and next-generation sequencing tools will allow for greater molecular insight into the mechanisms that underlie both the activity-dependent and -independent aspects of this process.
Acknowledgments
The authors would like to thank the previous authors of this chapter, S.M. Koch and E.M. Ullian, for foundational contributions to this work.
References
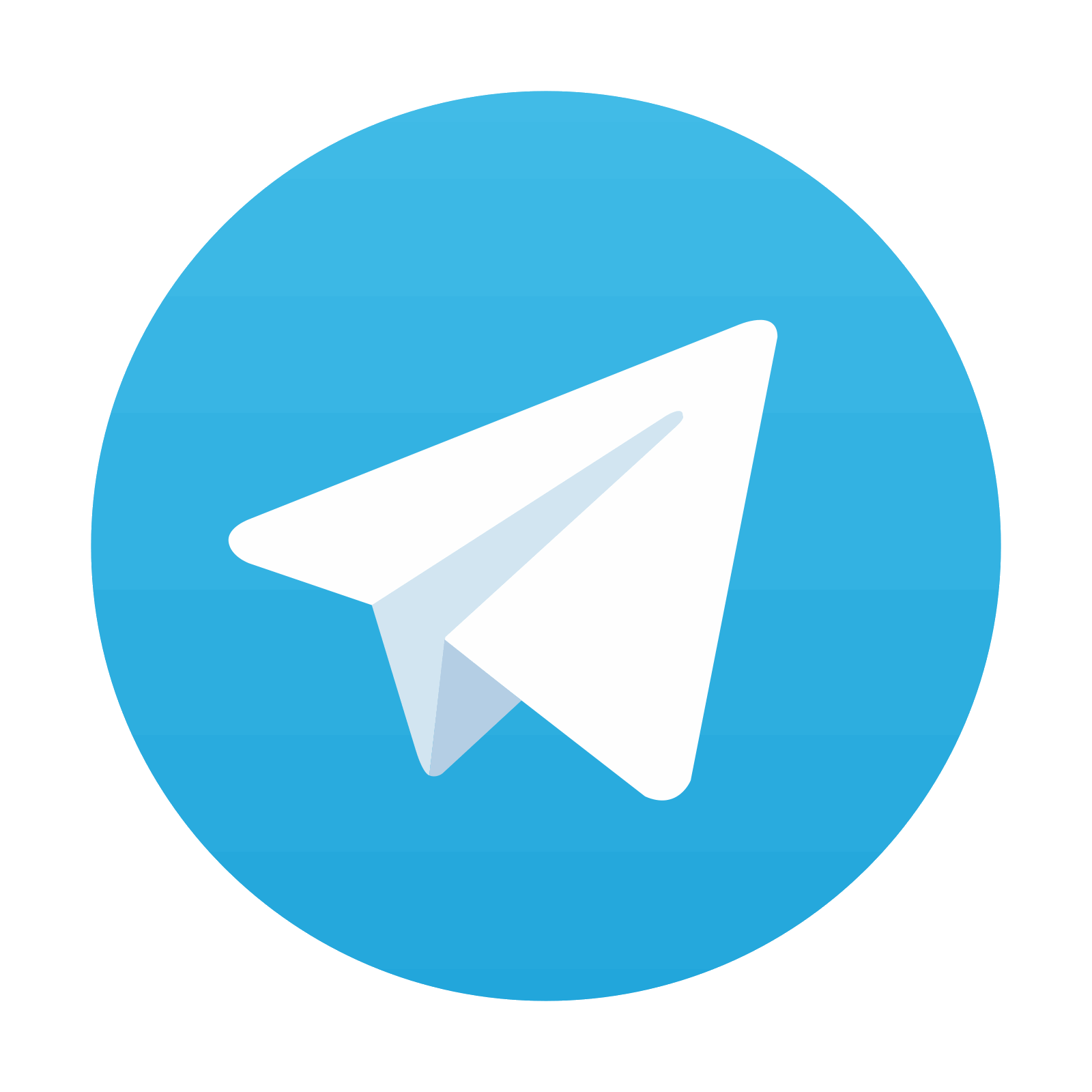
Stay updated, free articles. Join our Telegram channel
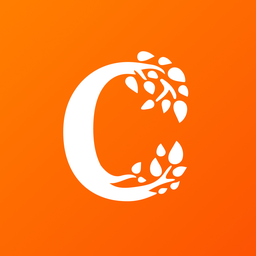
Full access? Get Clinical Tree
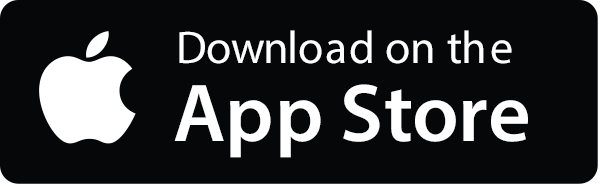
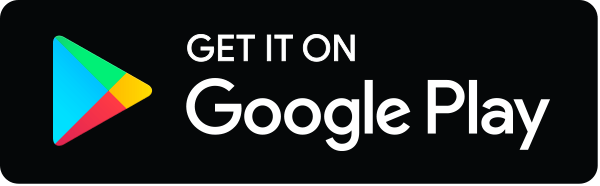
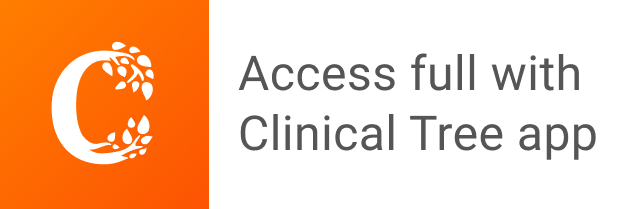