Introduction
A healthy cornea, together with the overlying tear film, is necessary to provide a proper anterior refractive surface and to protect the eye against infection and structural damage to the deeper components of the eye. The average adult cornea is 11.5–12 mm in horizontal diameter and about 1 mm smaller in vertical diameter. The anterior refractive power is +43.00 to +43.50 diopters (D). The shape of the cornea is prolate, being steeper centrally and flatter peripherally, which creates an aspherical optical system.
Embryology, Anatomy, and Physiology of the Cornea
Epithelium
The corneal epithelium is derived from surface ectoderm at approximately 5–6 weeks of gestation. It is composed of nonkeratinized, nonsecretory, stratified squamous epithelium ( Fig. 4.1.1 ), which is four- to six-cell-layers thick (40–50 µm). The epithelium is covered with a tear film of 7 µm thickness, which is optically important in masking microirregularities of the anterior epithelial surface. The tear–air interface, together with the underlying cornea, provides roughly two thirds of the total refractive power of the eye. The mucinous portion of tears, which forms the undercoat of the tear film and is produced by the conjunctival goblet cells, interacts closely with the corneal epithelial cell glycocalyx to allow hydrophilic spreading of the tear film with each blink of the eyelid. The tear film also helps protect the corneal surface from microbial invasion and from chemical, toxic, or foreign body damage. Thus, the ocular surface tear film and the corneal epithelium share an intimate mutual relationship, both anatomically and physiologically.

Corneal epithelial cells undergo orderly involution, apoptosis, and desquamation. Complete turnover of corneal epithelial cells occurs in about 7–10 days, with the deeper cells eventually replacing the desquamating superficial cells in an apically directed fashion. The most superficial cells of the corneal epithelium form an average of two to three layers of flat, polygonal cells. Extensive apical microvilli and microplicae characterize the cell membranes of the superficial cells, which, in turn, are covered by a fine, closely apposed, charged glycocalyceal layer. The apical membrane projections increase the surface area of contact and adherence between the tear film’s mucinous undercoat and the cell membrane. Laterally, adjacent superficial cells are joined by barrier tight-junctional complexes, which restrict entry of tears into the intercellular spaces. Thus a healthy epithelial surface repels dyes, such as fluorescein and rose bengal.
Beneath the superficial cell layer are the suprabasal or wing cells, so named for their cross-sectional alar shapes. This layer is about two to three cells deep and consists of cells that are less flat than the overlying superficial cells but possess similar tight, lateral, intercellular junctions. Beneath the wing cells are the basal cells, the deepest cellular layer of the corneal epithelium. The basal cell layer is composed of a single-cell layer of columnar epithelium approximately 20 µm tall. Besides the stem cells and transient amplifying cells, basal cells are the only corneal epithelial cells capable of mitosis. They are the source of both wing and superficial cells and possess lateral intercellular junctions characterized by gap junctions and zonulae adherens. The basal cells are attached to the underlying basement membrane by an extensive basal hemi-desmosomal system. This attachment is of pivotal importance in preventing the detachment of the multilayer epithelial sheet from the cornea. Abnormalities in this bonding system may result clinically in either recurrent corneal erosion syndromes or in persistent, nonhealing epithelial defects.
The basement membrane is composed of an extracellular matrix material secreted by the basal cells. Following destruction of the basement membrane, about 6 weeks are required for it to reconstitute and heal. The epithelial bond to the underlying, newly laid basement membrane tends to be unstable and weak during this period. The epithelium also adheres relatively poorly to bare stroma or Bowman’s layer. Under ordinary conditions, type IV collagen and laminin are the major components of the basement membrane; however, fibronectin production increases to high levels during acute epithelial injury. The basement membrane, approximately 0.05 µm in thickness, adheres to the underlying Bowman’s membrane through a poorly understood mechanism that involves the anchoring fibrils and plaques.
Epithelial stem cells—undifferentiated pluripotent cells that serve as an important source of new corneal epithelium—have been localized to the limbal basal epithelium. As the cells migrate to the central cornea, they differentiate into transient amplifying cells (cells capable of multiple but limited cellular division) and basal cells. The corneal epithelial cell layer mass appears to be the complex resultant of three phenomena. According to the “X, Y, Z hypothesis,” X is the proliferation of basal epithelial cells, Y is the centripetal mass movement of peripheral epithelial cells, and Z is the cell loss resulting from death and desquamation. These three phenomena probably are not totally independent of each other but, rather, are controlled by a complex interactive feedback mechanism that maintains the status quo, vis-à-vis cell density, cell distribution and polarity, and cell layer thickness. These cytodynamics are likely to be responsible for the striking verticillate (vortex or whorl-like) biochemical deposition patterns seen in Fabry’s disease ( Fig. 4.1.2 ) and drug deposition keratopathies (e.g., from chloroquine and amiodarone). Langerhans’ cells, immunologically active dendritic macrophages derived from bone marrow and capable of antigen processing, are present in the peripheral corneal epithelium near the limbus. Under certain conditions (e.g., corneal graft rejection or injury), these cells are found among the central corneal epithelial cells. Human lymphocyte antigens are expressed by these corneal Langerhans’ cells. Langerhans’ cells have been detected in the epithelial basal cell layer and in Bowman’s membrane in pathological inflammatory conditions, such as Thygeson’s superficial punctate keratitis. After treatment with topical corticosteroids, these cells are no longer detectable by laser confocal microscopy.

Stroma
In week 7 of gestation, after the establishment of the primitive endothelium, a second wave of neural crest cells forms the early corneal stroma. Akin to the dermis of the skin, the corneal stroma provides important structural integrity and comprises roughly 90% of the corneal thickness. The stroma differs from other collagenous structures in its transparency and biomechanical properties. These functional properties result from the precise organization of stromal fibers and extracellular matrix, and the relatively dehydrated state of the corneal stroma. The fibers are aligned in a parallel fashion within each lamella, and arranged at angles relative to fibers in adjacent lamellae. This network reduces forward light scatter and contributes to the mechanical strength of the cornea. The peripheral stroma is thicker than the central stroma and the collagen fibrils may change direction to run circumferentially as they approach the limbus. Bowman’s membrane is the acellular condensate of the most anterior portion of the stroma.
The stromal collagen fibrils, which provide the major tensile strength to the cornea, are composed mostly of type I collagen, but require a heterodimeric complex with type V collagen to obtain their unique and narrow diameter. They are surrounded by specialized proteoglycans, consisting of keratan sulfate or chondroitin sulfate/dermatan sulfate side chains, which help regulate hydration and structural properties. Keratocytes, the major cell type of the stroma, comprise approximately 10% of the stroma by volume and are involved in maintaining the extracellular matrix environment. More keratocytes are situated in the anterior stroma than in the posterior stroma. Morphological differences between the anterior and posterior stromal keratocytes, such as fenestrations, have been identified. Corneal “crystallins,” representing 25%–30% of soluble protein in keratocytes, appear to be responsible for reducing backscatter of light from the keratocytes and maintaining corneal transparency.
Dua et al. have proposed unique biomechanical properties of the most posterior 6–15 microns of the stroma (pre-Descemet’s layer [PDL] ). These five to eight lamellae of compact collagen appear distinct on electron microscopy and may be of relevance as a plane of cleavage in keratoplasty. The electron microscopic details of this membrane were first illustrated in 1972 by Fine and Yanoff.
Corneal shape and curvature are governed by the intrinsic biomechanical structure and extrinsic environment ( Fig. 4.1.3 ). Anterior corneal stromal rigidity in particular appears to be important in maintaining the corneal curvature. Organizational differences in the collagen bundles of the anterior stroma may contribute to a tighter cohesive strength in this area and may explain why the anterior curvature resists change to stromal hydration much more compared with the posterior stroma, which tends to develop folds more easily. Corneal nerves and sensation are derived from the nasociliary branch of the first (ophthalmic) division of the trigeminal nerve. In the superficial cornea, the nerves enter the stroma radially in thick trunks forming plexiform arrangements, which eventually perforate Bowman’s membrane to provide a rich plexus beneath the basal epithelial layer. The fibers appear to directly communicate with keratocytes and epithelial cells and may play an important role in corneal homeostasis.

Endothelium
In early embryogenesis, the posterior cornea is lined with a neural crest-derived monolayer of orderly arranged cuboidal cells. By the 78-mm stage, the cells become flattened and tightly abut one another. At this stage, immediately anterior to the flattened layer is a discontinuous, homogeneous acellular layer, which in time becomes Descemet’s membrane. By the 120-mm and 165-mm stages of development, the endothelial monolayer is uniform in thickness, spans the entire posterior corneal surface, and fuses with the cells of the trabecular meshwork. Similarly, Descemet’s membrane becomes continuous and uniform, fusing peripherally with the trabecular beams. The fusion site, known as Schwalbe’s line, is a gonioscopic landmark that defines the end of Descemet’s membrane and the start of the trabecular meshwork. At birth, the endothelium is approximately 10 µm thick.
The intact human endothelium is a monolayer, which appears as a honeycomb-like mosaic when viewed “en face” ( Fig. 4.1.4 ). The individual cells continue to flatten over time and stabilize at about 4 µm in thickness in adulthood ( Fig. 4.1.5 ). The posterior surface of the endothelium is devoid of villi, except in certain pathological conditions, in which it may develop epithelioid characteristics. Adjacent cells share extensive lateral interdigitations and possess gap and tight junctions along their lateral borders. The lateral membranes contain a high density of sodium (Na+), potassium (K+)–adenosine triphosphatase (ATPase) pump sites. The basal surface of the endothelium contains numerous hemi-desmosomes that promote adhesion to Descemet’s membrane. Endothelial cells contain numerous mitochondria and a prominent Golgi apparatus, and continuously secrete Descemet’s membrane throughout life, beginning in utero at the week 8 stage. The anterior portion of Descemet’s membrane formed in utero has a distinctive banded appearance when viewed by electron microscopy, but Descemet’s membrane produced after birth is unbanded and has an amorphous ultrastructural texture. This membrane is approximately 3 µm thick at birth, but thickens to 10 µm with age. Endothelial cell density and topography continue to change throughout life. From the second to eighth decades of life, the cell density declines from approximately 3000–4000 cells/mm 2 to around 2600 cells/mm 2 , and the percentage of hexagonal cells declines from about 75% to around 60%. The central endothelial cell density decreases at an average rate of 0.3% per year in normal corneas.


As a result of endothelial activity, the stroma is maintained in a relatively deturgesced state (78% water content). One hypothesis is that this endothelial activity is mediated by a pump–leak process; net fluid egress from the corneal stroma follows movement down an osmotic gradient from a relatively hypo-osmotic stroma toward a relatively hypertonic aqueous humor. This passive bulk fluid movement requires no energy. The energy-requiring processes are the intracellular and membrane-bound ion transport systems, which generate the osmotic gradient. The two most important ion transport systems are the membrane-bound Na + , K + -ATPase sites and the intracellular carbonic anhydrase pathway. Activity in both these pathways produces a net flux from stroma to aqueous humor. The barrier portion of the endothelium is unique in that it is permeable to some degree, permitting the ion flux necessary to establish the osmotic gradient.
Little in vivo mitotic potential exists within the normal endothelium but may come into play in pathological situations. Although the exact minimum number of cells per millimeter squared required to maintain corneal deturgescence is not known, corneas with central cell counts below 500 cells/mm 2 may be at risk for development of corneal edema. Endothelial cell morphology (size and shape) appears to correlate with pump function. An increase in cell size (polymegathism) and an increase in variation of cell shape (pleomorphism) correlate to reduced ability of the endothelial cells to deturgesce the cornea.
In vivo assessment of endothelial function relies on measurements of corneal thickness or on clinical morphological studies of the endothelial monolayer with specular microscopy. Measurement of the corneal thickness (pachymetry) indirectly reflects endothelial function. The average central corneal thickness is around 0.5 mm and gradually increases toward the periphery to around 0.7 mm. Normally, as a diurnal variation, corneas tend to be slightly thicker just after the person awakes in the morning. This increase in thickness is the consequence of diminished evaporation of water from underneath the closed eyelids, and the result of reduced nocturnal metabolic activity of the endothelium. Such overnight corneal swelling is more exaggerated in persons with unhealthy endothelium, causing blurred vision in the morning, but it gradually resolves later during the day.
Endothelial Responses to Stress
Mild endothelial stress may result in cell size and shape changes, whereas greater stress may result in cell loss as well as irreversible alterations in the endothelial cytoskeleton. Sources of stress may include metabolic disorders (hypoxia or hyperglycemia), toxins (drugs or their preservatives), injury (trauma or surgery), or alterations in pH or osmolarity. For example, contact lenses cause a hypoxic stress of varying degree to the endothelium. Over time, this may result in alteration of the morphology, microanatomy, and possibly the function of the endothelium. Hyperglycemia is another common metabolic stress that may produce changes in the endothelium. When compared with age-matched controls, the corneal endothelium in patients with type 1 and type 2 diabetes has a lower mean cell density and greater pleomorphism and polymegathism.
Tissue manipulation, fluid flow in the anterior chamber, and intracameral pharmacological agents introduced during anterior segment surgery may cause damage to the endothelium. Ophthalmic viscoelastic materials (composed of hydroxypropyl methylcellulose, chondroitin sulfate, or sodium hyaluronate) provide significant protection against intraoperative trauma to the endothelium.
Glaucoma has been associated with endothelial cell loss. Compared with age-matched controls, significantly lower endothelial cell counts were noted in patients with glaucoma and ocular hypertension in one study. Cell counts were inversely proportional to the mean intraocular pressure in the glaucoma and ocular hypertension groups. Mechanisms of cell loss may include direct damage from intraocular pressure, congenital alterations of endothelium in glaucoma, and drug toxicity.
Introduction
A healthy cornea, together with the overlying tear film, is necessary to provide a proper anterior refractive surface and to protect the eye against infection and structural damage to the deeper components of the eye. The average adult cornea is 11.5–12 mm in horizontal diameter and about 1 mm smaller in vertical diameter. The anterior refractive power is +43.00 to +43.50 diopters (D). The shape of the cornea is prolate, being steeper centrally and flatter peripherally, which creates an aspherical optical system.
Embryology, Anatomy, and Physiology of the Cornea
Epithelium
The corneal epithelium is derived from surface ectoderm at approximately 5–6 weeks of gestation. It is composed of nonkeratinized, nonsecretory, stratified squamous epithelium ( Fig. 4.1.1 ), which is four- to six-cell-layers thick (40–50 µm). The epithelium is covered with a tear film of 7 µm thickness, which is optically important in masking microirregularities of the anterior epithelial surface. The tear–air interface, together with the underlying cornea, provides roughly two thirds of the total refractive power of the eye. The mucinous portion of tears, which forms the undercoat of the tear film and is produced by the conjunctival goblet cells, interacts closely with the corneal epithelial cell glycocalyx to allow hydrophilic spreading of the tear film with each blink of the eyelid. The tear film also helps protect the corneal surface from microbial invasion and from chemical, toxic, or foreign body damage. Thus, the ocular surface tear film and the corneal epithelium share an intimate mutual relationship, both anatomically and physiologically.

Corneal epithelial cells undergo orderly involution, apoptosis, and desquamation. Complete turnover of corneal epithelial cells occurs in about 7–10 days, with the deeper cells eventually replacing the desquamating superficial cells in an apically directed fashion. The most superficial cells of the corneal epithelium form an average of two to three layers of flat, polygonal cells. Extensive apical microvilli and microplicae characterize the cell membranes of the superficial cells, which, in turn, are covered by a fine, closely apposed, charged glycocalyceal layer. The apical membrane projections increase the surface area of contact and adherence between the tear film’s mucinous undercoat and the cell membrane. Laterally, adjacent superficial cells are joined by barrier tight-junctional complexes, which restrict entry of tears into the intercellular spaces. Thus a healthy epithelial surface repels dyes, such as fluorescein and rose bengal.
Beneath the superficial cell layer are the suprabasal or wing cells, so named for their cross-sectional alar shapes. This layer is about two to three cells deep and consists of cells that are less flat than the overlying superficial cells but possess similar tight, lateral, intercellular junctions. Beneath the wing cells are the basal cells, the deepest cellular layer of the corneal epithelium. The basal cell layer is composed of a single-cell layer of columnar epithelium approximately 20 µm tall. Besides the stem cells and transient amplifying cells, basal cells are the only corneal epithelial cells capable of mitosis. They are the source of both wing and superficial cells and possess lateral intercellular junctions characterized by gap junctions and zonulae adherens. The basal cells are attached to the underlying basement membrane by an extensive basal hemi-desmosomal system. This attachment is of pivotal importance in preventing the detachment of the multilayer epithelial sheet from the cornea. Abnormalities in this bonding system may result clinically in either recurrent corneal erosion syndromes or in persistent, nonhealing epithelial defects.
The basement membrane is composed of an extracellular matrix material secreted by the basal cells. Following destruction of the basement membrane, about 6 weeks are required for it to reconstitute and heal. The epithelial bond to the underlying, newly laid basement membrane tends to be unstable and weak during this period. The epithelium also adheres relatively poorly to bare stroma or Bowman’s layer. Under ordinary conditions, type IV collagen and laminin are the major components of the basement membrane; however, fibronectin production increases to high levels during acute epithelial injury. The basement membrane, approximately 0.05 µm in thickness, adheres to the underlying Bowman’s membrane through a poorly understood mechanism that involves the anchoring fibrils and plaques.
Epithelial stem cells—undifferentiated pluripotent cells that serve as an important source of new corneal epithelium—have been localized to the limbal basal epithelium. As the cells migrate to the central cornea, they differentiate into transient amplifying cells (cells capable of multiple but limited cellular division) and basal cells. The corneal epithelial cell layer mass appears to be the complex resultant of three phenomena. According to the “X, Y, Z hypothesis,” X is the proliferation of basal epithelial cells, Y is the centripetal mass movement of peripheral epithelial cells, and Z is the cell loss resulting from death and desquamation. These three phenomena probably are not totally independent of each other but, rather, are controlled by a complex interactive feedback mechanism that maintains the status quo, vis-à-vis cell density, cell distribution and polarity, and cell layer thickness. These cytodynamics are likely to be responsible for the striking verticillate (vortex or whorl-like) biochemical deposition patterns seen in Fabry’s disease ( Fig. 4.1.2 ) and drug deposition keratopathies (e.g., from chloroquine and amiodarone). Langerhans’ cells, immunologically active dendritic macrophages derived from bone marrow and capable of antigen processing, are present in the peripheral corneal epithelium near the limbus. Under certain conditions (e.g., corneal graft rejection or injury), these cells are found among the central corneal epithelial cells. Human lymphocyte antigens are expressed by these corneal Langerhans’ cells. Langerhans’ cells have been detected in the epithelial basal cell layer and in Bowman’s membrane in pathological inflammatory conditions, such as Thygeson’s superficial punctate keratitis. After treatment with topical corticosteroids, these cells are no longer detectable by laser confocal microscopy.

Stroma
In week 7 of gestation, after the establishment of the primitive endothelium, a second wave of neural crest cells forms the early corneal stroma. Akin to the dermis of the skin, the corneal stroma provides important structural integrity and comprises roughly 90% of the corneal thickness. The stroma differs from other collagenous structures in its transparency and biomechanical properties. These functional properties result from the precise organization of stromal fibers and extracellular matrix, and the relatively dehydrated state of the corneal stroma. The fibers are aligned in a parallel fashion within each lamella, and arranged at angles relative to fibers in adjacent lamellae. This network reduces forward light scatter and contributes to the mechanical strength of the cornea. The peripheral stroma is thicker than the central stroma and the collagen fibrils may change direction to run circumferentially as they approach the limbus. Bowman’s membrane is the acellular condensate of the most anterior portion of the stroma.
The stromal collagen fibrils, which provide the major tensile strength to the cornea, are composed mostly of type I collagen, but require a heterodimeric complex with type V collagen to obtain their unique and narrow diameter. They are surrounded by specialized proteoglycans, consisting of keratan sulfate or chondroitin sulfate/dermatan sulfate side chains, which help regulate hydration and structural properties. Keratocytes, the major cell type of the stroma, comprise approximately 10% of the stroma by volume and are involved in maintaining the extracellular matrix environment. More keratocytes are situated in the anterior stroma than in the posterior stroma. Morphological differences between the anterior and posterior stromal keratocytes, such as fenestrations, have been identified. Corneal “crystallins,” representing 25%–30% of soluble protein in keratocytes, appear to be responsible for reducing backscatter of light from the keratocytes and maintaining corneal transparency.
Dua et al. have proposed unique biomechanical properties of the most posterior 6–15 microns of the stroma (pre-Descemet’s layer [PDL] ). These five to eight lamellae of compact collagen appear distinct on electron microscopy and may be of relevance as a plane of cleavage in keratoplasty. The electron microscopic details of this membrane were first illustrated in 1972 by Fine and Yanoff.
Corneal shape and curvature are governed by the intrinsic biomechanical structure and extrinsic environment ( Fig. 4.1.3 ). Anterior corneal stromal rigidity in particular appears to be important in maintaining the corneal curvature. Organizational differences in the collagen bundles of the anterior stroma may contribute to a tighter cohesive strength in this area and may explain why the anterior curvature resists change to stromal hydration much more compared with the posterior stroma, which tends to develop folds more easily. Corneal nerves and sensation are derived from the nasociliary branch of the first (ophthalmic) division of the trigeminal nerve. In the superficial cornea, the nerves enter the stroma radially in thick trunks forming plexiform arrangements, which eventually perforate Bowman’s membrane to provide a rich plexus beneath the basal epithelial layer. The fibers appear to directly communicate with keratocytes and epithelial cells and may play an important role in corneal homeostasis.

Endothelium
In early embryogenesis, the posterior cornea is lined with a neural crest-derived monolayer of orderly arranged cuboidal cells. By the 78-mm stage, the cells become flattened and tightly abut one another. At this stage, immediately anterior to the flattened layer is a discontinuous, homogeneous acellular layer, which in time becomes Descemet’s membrane. By the 120-mm and 165-mm stages of development, the endothelial monolayer is uniform in thickness, spans the entire posterior corneal surface, and fuses with the cells of the trabecular meshwork. Similarly, Descemet’s membrane becomes continuous and uniform, fusing peripherally with the trabecular beams. The fusion site, known as Schwalbe’s line, is a gonioscopic landmark that defines the end of Descemet’s membrane and the start of the trabecular meshwork. At birth, the endothelium is approximately 10 µm thick.
The intact human endothelium is a monolayer, which appears as a honeycomb-like mosaic when viewed “en face” ( Fig. 4.1.4 ). The individual cells continue to flatten over time and stabilize at about 4 µm in thickness in adulthood ( Fig. 4.1.5 ). The posterior surface of the endothelium is devoid of villi, except in certain pathological conditions, in which it may develop epithelioid characteristics. Adjacent cells share extensive lateral interdigitations and possess gap and tight junctions along their lateral borders. The lateral membranes contain a high density of sodium (Na+), potassium (K+)–adenosine triphosphatase (ATPase) pump sites. The basal surface of the endothelium contains numerous hemi-desmosomes that promote adhesion to Descemet’s membrane. Endothelial cells contain numerous mitochondria and a prominent Golgi apparatus, and continuously secrete Descemet’s membrane throughout life, beginning in utero at the week 8 stage. The anterior portion of Descemet’s membrane formed in utero has a distinctive banded appearance when viewed by electron microscopy, but Descemet’s membrane produced after birth is unbanded and has an amorphous ultrastructural texture. This membrane is approximately 3 µm thick at birth, but thickens to 10 µm with age. Endothelial cell density and topography continue to change throughout life. From the second to eighth decades of life, the cell density declines from approximately 3000–4000 cells/mm 2 to around 2600 cells/mm 2 , and the percentage of hexagonal cells declines from about 75% to around 60%. The central endothelial cell density decreases at an average rate of 0.3% per year in normal corneas.


As a result of endothelial activity, the stroma is maintained in a relatively deturgesced state (78% water content). One hypothesis is that this endothelial activity is mediated by a pump–leak process; net fluid egress from the corneal stroma follows movement down an osmotic gradient from a relatively hypo-osmotic stroma toward a relatively hypertonic aqueous humor. This passive bulk fluid movement requires no energy. The energy-requiring processes are the intracellular and membrane-bound ion transport systems, which generate the osmotic gradient. The two most important ion transport systems are the membrane-bound Na + , K + -ATPase sites and the intracellular carbonic anhydrase pathway. Activity in both these pathways produces a net flux from stroma to aqueous humor. The barrier portion of the endothelium is unique in that it is permeable to some degree, permitting the ion flux necessary to establish the osmotic gradient.
Little in vivo mitotic potential exists within the normal endothelium but may come into play in pathological situations. Although the exact minimum number of cells per millimeter squared required to maintain corneal deturgescence is not known, corneas with central cell counts below 500 cells/mm 2 may be at risk for development of corneal edema. Endothelial cell morphology (size and shape) appears to correlate with pump function. An increase in cell size (polymegathism) and an increase in variation of cell shape (pleomorphism) correlate to reduced ability of the endothelial cells to deturgesce the cornea.
In vivo assessment of endothelial function relies on measurements of corneal thickness or on clinical morphological studies of the endothelial monolayer with specular microscopy. Measurement of the corneal thickness (pachymetry) indirectly reflects endothelial function. The average central corneal thickness is around 0.5 mm and gradually increases toward the periphery to around 0.7 mm. Normally, as a diurnal variation, corneas tend to be slightly thicker just after the person awakes in the morning. This increase in thickness is the consequence of diminished evaporation of water from underneath the closed eyelids, and the result of reduced nocturnal metabolic activity of the endothelium. Such overnight corneal swelling is more exaggerated in persons with unhealthy endothelium, causing blurred vision in the morning, but it gradually resolves later during the day.
Endothelial Responses to Stress
Mild endothelial stress may result in cell size and shape changes, whereas greater stress may result in cell loss as well as irreversible alterations in the endothelial cytoskeleton. Sources of stress may include metabolic disorders (hypoxia or hyperglycemia), toxins (drugs or their preservatives), injury (trauma or surgery), or alterations in pH or osmolarity. For example, contact lenses cause a hypoxic stress of varying degree to the endothelium. Over time, this may result in alteration of the morphology, microanatomy, and possibly the function of the endothelium. Hyperglycemia is another common metabolic stress that may produce changes in the endothelium. When compared with age-matched controls, the corneal endothelium in patients with type 1 and type 2 diabetes has a lower mean cell density and greater pleomorphism and polymegathism.
Tissue manipulation, fluid flow in the anterior chamber, and intracameral pharmacological agents introduced during anterior segment surgery may cause damage to the endothelium. Ophthalmic viscoelastic materials (composed of hydroxypropyl methylcellulose, chondroitin sulfate, or sodium hyaluronate) provide significant protection against intraoperative trauma to the endothelium.
Glaucoma has been associated with endothelial cell loss. Compared with age-matched controls, significantly lower endothelial cell counts were noted in patients with glaucoma and ocular hypertension in one study. Cell counts were inversely proportional to the mean intraocular pressure in the glaucoma and ocular hypertension groups. Mechanisms of cell loss may include direct damage from intraocular pressure, congenital alterations of endothelium in glaucoma, and drug toxicity.
Embryology, Anatomy, and Physiology of the Cornea
Epithelium
The corneal epithelium is derived from surface ectoderm at approximately 5–6 weeks of gestation. It is composed of nonkeratinized, nonsecretory, stratified squamous epithelium ( Fig. 4.1.1 ), which is four- to six-cell-layers thick (40–50 µm). The epithelium is covered with a tear film of 7 µm thickness, which is optically important in masking microirregularities of the anterior epithelial surface. The tear–air interface, together with the underlying cornea, provides roughly two thirds of the total refractive power of the eye. The mucinous portion of tears, which forms the undercoat of the tear film and is produced by the conjunctival goblet cells, interacts closely with the corneal epithelial cell glycocalyx to allow hydrophilic spreading of the tear film with each blink of the eyelid. The tear film also helps protect the corneal surface from microbial invasion and from chemical, toxic, or foreign body damage. Thus, the ocular surface tear film and the corneal epithelium share an intimate mutual relationship, both anatomically and physiologically.

Corneal epithelial cells undergo orderly involution, apoptosis, and desquamation. Complete turnover of corneal epithelial cells occurs in about 7–10 days, with the deeper cells eventually replacing the desquamating superficial cells in an apically directed fashion. The most superficial cells of the corneal epithelium form an average of two to three layers of flat, polygonal cells. Extensive apical microvilli and microplicae characterize the cell membranes of the superficial cells, which, in turn, are covered by a fine, closely apposed, charged glycocalyceal layer. The apical membrane projections increase the surface area of contact and adherence between the tear film’s mucinous undercoat and the cell membrane. Laterally, adjacent superficial cells are joined by barrier tight-junctional complexes, which restrict entry of tears into the intercellular spaces. Thus a healthy epithelial surface repels dyes, such as fluorescein and rose bengal.
Beneath the superficial cell layer are the suprabasal or wing cells, so named for their cross-sectional alar shapes. This layer is about two to three cells deep and consists of cells that are less flat than the overlying superficial cells but possess similar tight, lateral, intercellular junctions. Beneath the wing cells are the basal cells, the deepest cellular layer of the corneal epithelium. The basal cell layer is composed of a single-cell layer of columnar epithelium approximately 20 µm tall. Besides the stem cells and transient amplifying cells, basal cells are the only corneal epithelial cells capable of mitosis. They are the source of both wing and superficial cells and possess lateral intercellular junctions characterized by gap junctions and zonulae adherens. The basal cells are attached to the underlying basement membrane by an extensive basal hemi-desmosomal system. This attachment is of pivotal importance in preventing the detachment of the multilayer epithelial sheet from the cornea. Abnormalities in this bonding system may result clinically in either recurrent corneal erosion syndromes or in persistent, nonhealing epithelial defects.
The basement membrane is composed of an extracellular matrix material secreted by the basal cells. Following destruction of the basement membrane, about 6 weeks are required for it to reconstitute and heal. The epithelial bond to the underlying, newly laid basement membrane tends to be unstable and weak during this period. The epithelium also adheres relatively poorly to bare stroma or Bowman’s layer. Under ordinary conditions, type IV collagen and laminin are the major components of the basement membrane; however, fibronectin production increases to high levels during acute epithelial injury. The basement membrane, approximately 0.05 µm in thickness, adheres to the underlying Bowman’s membrane through a poorly understood mechanism that involves the anchoring fibrils and plaques.
Epithelial stem cells—undifferentiated pluripotent cells that serve as an important source of new corneal epithelium—have been localized to the limbal basal epithelium. As the cells migrate to the central cornea, they differentiate into transient amplifying cells (cells capable of multiple but limited cellular division) and basal cells. The corneal epithelial cell layer mass appears to be the complex resultant of three phenomena. According to the “X, Y, Z hypothesis,” X is the proliferation of basal epithelial cells, Y is the centripetal mass movement of peripheral epithelial cells, and Z is the cell loss resulting from death and desquamation. These three phenomena probably are not totally independent of each other but, rather, are controlled by a complex interactive feedback mechanism that maintains the status quo, vis-à-vis cell density, cell distribution and polarity, and cell layer thickness. These cytodynamics are likely to be responsible for the striking verticillate (vortex or whorl-like) biochemical deposition patterns seen in Fabry’s disease ( Fig. 4.1.2 ) and drug deposition keratopathies (e.g., from chloroquine and amiodarone). Langerhans’ cells, immunologically active dendritic macrophages derived from bone marrow and capable of antigen processing, are present in the peripheral corneal epithelium near the limbus. Under certain conditions (e.g., corneal graft rejection or injury), these cells are found among the central corneal epithelial cells. Human lymphocyte antigens are expressed by these corneal Langerhans’ cells. Langerhans’ cells have been detected in the epithelial basal cell layer and in Bowman’s membrane in pathological inflammatory conditions, such as Thygeson’s superficial punctate keratitis. After treatment with topical corticosteroids, these cells are no longer detectable by laser confocal microscopy.
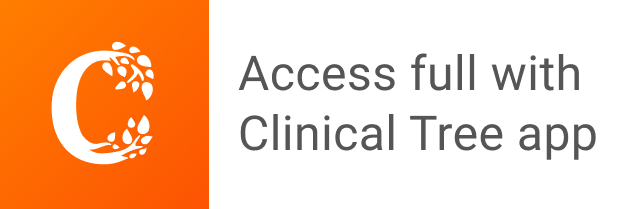