Introduction
The outermost, fibrous tunic of the human eye consists of the cornea and the sclera ( Fig. 4.1A,B ). Both are soft connective tissues that provide structural integrity to the globe and protect the inner components of the eye from physical injury. The clear, transparent cornea ( Fig. 4.1A,C ) covers the anterior one-sixth of the total surface area of the globe, and the white, opaque sclera ( Fig. 4.1A ) covers the remaining five-sixths. The cornea and the lens are the eye’s primary refractive structures and both have two key optical properties to this end—refractive power (light refraction) and transparency (light transmission). The presence of a healthy cornea is essential for good vision as it is basically the window of the eye. The cornea is most analogous to the external lens of a compound lens camera. By comparison, the sclera predominantly serves more of a biomechanical function and is analogous to the housing of the camera and lens.

The normal human cornea is between 500 and 650 microns (µm) thick and is arranged in five basic layers—epithelium, Bowman’s layer, stroma, Descemet’s membrane, and endothelium ( Fig. 4.1D ), each having distinctly different structural and functional characteristics. It also is composed of three major cell types—epithelial, stromal keratocyte, and endothelial cells. However, there are several other cell types found in the stroma, (e.g., Langerhans cells, nonmyelinated Schwann cells, and dendritic cells). Two of these, epithelium and endothelium, form cellular barrier layers to the stroma. Thus, their resistance to diffusion of solutes and bulk fluid flow are of considerable importance to maintaining normal corneal function (resistance to diffusion of solutes and fluid flow: epithelium [2000] >> endothelium [10] > stroma [1]). All three can replicate through mitosis, but vary considerably in their in vivo proliferative capacity, with epithelial cells having the highest rates of cell division and endothelial cells being the least renewable. This fact is seen clinically because epithelial cells can completely regenerate after injury (e.g., corneal abrasions), whereas endothelial cells, as a result of limited in vivo proliferation, are most commonly involved in age-related disease (e.g., Fuchs’ dystrophy) or injury-related disease (e.g., pseudophakic bullous keratopathy [PBK]), ultimately resulting in corneal edema and bullous keratopathy. However, more recently, an early proliferative progenitor endothelial cell has been described in the transition zone. Corneal stromal keratocytes are a middle ground compromise between these two extremes. One major disadvantage of the epithelium’s high proliferative potential is that it can occasionally go unchecked, resulting in cancer (e.g., squamous cell cancers of the cornea), whereas keratocytes and endothelial cells do not have this risk.
The sclera is 0.3- to 1.35-mm thick and is arranged into three layers—episclera, scleral stroma proper, and lamina fusca; each having distinctly different structural and functional characteristics. The sclera is composed of only one major cell type, the sclera fibrocyte, which has moderate proliferative potential, like that of the corneal stromal keratocyte. Because the sclera has no cellular barrier layers, its permeability properties are quite similar to those of the corneal stroma. The sclera is an excellent example of a tissue made for biomechanical stability, as it is stiff, strong, and tough. As such, disease of the sclera commonly results in loss of structural strength, abnormal size owing to dysregulated growth, and inflammatory conditions that commonly also affect the joints in the body. Many animals have a very rigid sclera that is often supported by bone or cartilage. Humans deviate from this extreme in that their sclera is a less rigid fibrous connective tissue, perhaps reflecting its need to maintain an even blood flow to the choroid and retina during large excursions in eye motility.
This chapter reviews and explains the structure and function of the cornea and sclera, providing a framework for understanding normal health and disease of each tissue with an emphasis on function.
Cornea
Embryology, growth, development, and aging in human eyes
Between 4 and 5 weeks’ gestation (27–36 days), surface ectodermal cells left behind after vesicle separation become the primitive, undifferentiated corneal epithelium, which is composed initially of two cell layers. The primitive corneal epithelium immediately produces a primary acellular corneal stroma, or postepithelial layer. This is seen as the gradual subepithelial addition of diagonal and then randomly oriented fibrillar elements, which later thicken into collagen fibrils that are slightly smaller in diameter than stromal collagen fibrils. The Bowman’s layer is thought to be a distinct, dense anterior-most remnant of this embryologic layer, which is first detectable by light microscopy around 20 weeks’ gestation. Around 12 weeks’ gestation, a time period between eyelid fusion at 8 weeks’ gestation and eyelid opening at 26 weeks’ gestation, the epithelium differentiates to become a stratified, squamous epithelium, four cell layers thick, which then produces an epithelial basement membrane. It remains four cell layers thick until approximately 6 months after birth when it reaches adult levels of four to six cell layers thick. Early in gestation, the epithelial basement membrane and anchoring complexes on the basal surface of the epithelium are absent. Rudimentary epithelial basement membrane and anchoring complexes only become detectable by 17 weeks’ gestation. With further development in utero, the thickness and number of these structures gradually increase.
A first wave of neural crest-derived mesenchymal cells begins to migrate beneath the corneal epithelial cells from the limbus around 5 weeks’ gestation (33 days); these cells form the primitive endothelium. The primitive endothelium is initially composed of two cell layers. By 8 weeks’ gestation, it becomes a monolayer that starts to produce Descemet’s membrane, which becomes recognizable on light microscopy at 3 to 4 months’ gestation. The epithelium and endothelium remain closely opposed until 7 weeks’ gestation (49 days), when a second wave of mesenchymal cells begins to migrate centrally from the limbus between the epithelium and endothelium invading below and into most of the primary acellular stroma. The cells do not enter the anterior-most 10 µm of stroma, which lacks keratocan, a proteoglycan core protein signal thought to be required for cellular invasion. This second wave of cells forms the stroma proper, or secondary cellular corneal stroma, as production of lamellar collagen begins within a few days in a posterior-to-anterior fashion. It is believed that the invading mesenchymal cells, destined to become keratocytes, use the primary acellular stroma as a scaffold, primarily in the anterior third of stroma proper. This concurs with the significant lamellar interweaving and oblique lamellar orientation in the anterior third of postnatal human corneal stroma, as well as each successive lamellar layer being rotated 1 to 2 degrees clockwise. This directional rotation is the same in both right and left eyes. In the posterior two-thirds of the stroma, the corneal stroma is composed of essentially orthogonal lamellae. By 3 months’ gestation, corneal nerves invade the stroma and eventually penetrate through the Bowman’s layer so that nerve endings develop in the epithelium. Studies also suggest that by 5 months’ gestation, tight junctions form around all the corneal endothelial cells and by 5 to 7 months’ gestation the in-utero cornea becomes transparent as the density of functioning endothelial Na + /K + -ATPase metabolic pump sites increases to adult levels. By 7 months’ gestation, the cornea resembles that of the adult in most structural characteristics other than size. At birth in the full-term infant, the horizontal corneal diameter is about 9.8 mm and the corneal surface area is around 102 mm 2 . The cornea of the newborn infant is approximately 75% to 80% of the size of the adult human cornea ( Fig. 4.2A–C ), whereas the posterior segment is less than 50% of adult size ( Fig. 4.2D ). At birth, the cross-sectional thickness of the four cell layer epithelium averages 50 µm, the Bowman’s layer averages 10 µm, the central stroma proper averages 500 µm, Descemet’s membrane averages 4 µm, and the endothelium averages 6-µm thick (total mean central corneal thickness ∼570 µm).

During infancy, the cornea continues to grow, reaching adult size around 2 years of age with a horizontal diameter of 11.7 mm, surface area of 138 mm 2 , anterior surface curvature of 44.1 diopters (D) ( Fig. 4.2A ), and mean central corneal thickness of 544 µm ( Fig. 4.2C ). Thereafter, it changes very little in size, shape, transparency, or curvature, although a shift from with-the-rule to against-the-rule astigmatism has been associated with aging ( Fig. 4.2B ). This means that if we consider the cornea as a sphere, “with-the-rule” astigmatism is where the curvature is steepest is near the 90-degree meridian, whereas “against-the-rule” is astigmatism in which the steepest curve lies near the 180-degree meridian ( Fig. 4.2B ). Postnatal aging is associated with several structural changes to the corneal tissue including: (1) epithelial basement membrane growth or thickening of an additional 100 to 300 nm or a rate of approximately 30 nm per decade of life; (2) decreased keratocyte, sub-basal nerve fiber, and endothelial cell density, presumably from stress-induced premature senescence; (3) increased stiffness, strength, and toughness of the stroma, from enzymatic maturation and nonenzymatic age-related glycation-induced crosslinking of collagen fibrils; (4) Descemet’s membrane thickening of an additional 6 to 11 µm or a rate of approximately 1 µm per decade of life; and (5) possible degeneration of extracellular matrix structures. These structural and cellular changes, however, minimally affect the optical and barrier functions of the cornea and perhaps improve its mechanical function. For example, corneal ectasia from natural causes such as keratoconus is rarely seen after 40 years of age. Only three documented functional consequences are associated with aging—impaired corneal wound healing, decreased corneal sensation, and decreased extensibility of its tissue. In elderly individuals or younger individuals with lipid abnormalities, the cornea often becomes yellowish in the periphery of the cornea due to a fine deposition of lipid. This condition is called arcus senilis.
Major corneal reference points and measurements
When viewed anteriorly in the living eye, the adult human cornea appears elliptical ( Fig. 4.3A ), as the largest diameter is typically in the horizontal meridian (mean 11.7 mm) and the smallest is in the vertical meridian (mean 10.6 mm). This elliptical configuration is brought about by anterior extension of the opaque sclera superiorly and inferiorly. When viewed from the posterior surface, the cornea is actually circular ( Fig. 4.3A ), with an average horizontal and vertical diameter of 11.7 mm. The average radius of curvature of the anterior and posterior corneal surface is 7.8 mm and 6.5 mm, respectively, which is significantly less than the 11.5-mm average radius of curvature of the sclera. This results in a small 1.5- to 2-mm transition zone that forms an external and internal surface groove, or scleral sulcus, where the steeper cornea meets the flatter sclera ( Fig. 4.3A ). This sulcus typically is not obvious clinically because it is filled in by overlying episclera and conjunctiva externally. The tissue in this transition zone is known as the limbus ( Fig. 4.3B ), which averages 1.5-mm wide in the horizontal meridian and 2-mm wide in the vertical meridian. It is important because it contains adult corneal stem cell populations, contains the trabecular meshwork, which is the conventional outflow pathway for the aqueous humor, and is the inciting site of pathology in a few immunologic diseases. The limbus is also a major anatomic reference point for planning surgical entry into the anterior segment because it appears clinically as a blue transition zone. Therefore, an incision placed anterior to the blue zone is anatomically in the peripheral cornea, safely inside the trabecular meshwork and stem cells. The cornea is thinner in the center, measuring on average 544 ± 34 µm (range: 440–650 µm) with ultrasound-based corneal thickness measurements (ultrasound pachymetry), and increasing in thickness in the periphery to approximately 700 µm as it reaches the limbus. A meta-analysis of all cross-sectional and longitudinal corneal thickness studies over a 30-year time period showed that no significant age-related change in central corneal thickness occurred beyond the infant years.

The central cornea is overlying the entrance pupil, which is a virtual image of the real pupil and is typically located 0.5-mm anterior to, and is 14% larger than, the real pupil. It contains the central or effective optical zone of the cornea ( Fig. 4.3C ). The central optical zone is the portion of the cornea that can successfully refract a cone-like bundle of light from a distant or near fixation target through the pupil of the eye, and it then directly refracts it onto the fovea. The central location and size of this central optical zone dynamically changes according to the location of the fixation target in relation to the cornea (e.g., more distant fixation target = larger-diameter central optical zone, off-center fixation target = off-center central optical zone) and the aperture of the real pupil in various lighting conditions. This is because the bundle of light has the same cross-sectional shape as the pupil (e.g., an oval pupil results in an oval central optical zone) and its diameter is defined by the pupil’s diameter, as well as the location of fixation target. The central optical zone’s diameter typically averages 3.6 ± 0.8 mm in photopic lighting and 5.8 ± 0.9 mm in scotopic light, with a range between 1.5 and 9.0 mm depending on the lighting condition. The remaining cornea peripheral to this central optical zone is the peripheral optical zone, which can refract light through the entrance pupil; however, it does so at such an acute angle that it only affects the more peripheral aspects of the retina ( Fig. 4.3C )—it rarely directly impacts foveal vision.
Within the central optical zone are three major corneal reference points ( Fig. 4.3D ) that are extremely useful in determining the shape, refractive power, and biomechanical properties of the cornea, as they are statically fixed reference points. The first is called the corneal apex; it is defined as the steepest point or area of the cornea. Its exact location on the cornea is referenced in relation to the corneal intercept of an imaging device’s optical axis ( Fig. 4.3E ). The corneal intercept of the imaging device’s optical axis is termed the device’s axis point (DAP). On average, the corneal apex is usually located 0.8-mm temporal and 0.2-mm superior to the DAP or 0.5-mm temporal and 0.5-mm superior to the corneal intercept of the line of sight of the eye ( Fig. 4.3D ), but considerable interindividual variability is found in the location of the corneal apex in comparison to the average. The clinical utility of the corneal apex is that it is of paramount importance in the selection and fitting of contact lenses and in determining the geometric aspheric shape of the cornea. Significant decentration of the corneal apex from the DAP may give a false interpretation of corneal shape (e.g., asymmetric shape). The easiest way to clarify whether this is real or due to artifact is to aim the imaging device’s optical axis directly at the corneal apex, which is more cumbersome and difficult to do than using a standard alignment position.
The second major corneal reference point is the corneal intercept of the line of sight, also known as the corneal sighting center (CSC). The line of sight is an actual principal axis of the real eye as opposed to a theoretical construct of a schematic eye (e.g., visual axis), and it is defined as the principal axis joining a distant fixation point to the fovea. Theoretically, it is always thought to cross the center of the entrance pupil, which may not be true in the real eye because it is known that the center of the entrance pupil dynamically and unpredictably shifts up to 0.7 mm in direction with changes in pupil diameter, whereas the line of sight is a statically fixed axis line. Thus, the line of sight is perhaps best thought of as a line that connects the fixation target to the CSC on the anterior surface of the cornea and then, via an unknown nonlinear pathway, is refracted in the cornea and by the lens to focus on the fovea. It is of utmost importance to know the location of the line of sight and CSC in certain clinical situations, to obtain the best visual results with keratorefractive surgery. This is particularly so with retreatments and customized ablations, and for calculating the proper posterior chamber intraocular lens (PCIOL) power to put in during cataract extraction (CE), especially after previous refractive surgery or with anterior surface irregularities. As subclinical decentrations 0.5 mm (or more) or torsional misalignments 15 degrees (or more) can yield unwanted visual symptoms (e.g., coma or other higher-order aberrations [HOAs]). On average, the CSC is 0.4-mm nasal and 0.3-mm superior to the dynamically, unfixed pupillary axis or 0.5-mm inferior and 0.5-mm nasal to the static, fixed corneal apex reference point ( Fig. 4.3D ), but its location overall is highly variable between different individuals. Using a keratographer, topographer, or tomographer in nonstandard alignment position, the CSC can be directly determined by having the patient directly look at a luminous fixation point centered in the circular rings of the imaging device. It is then marked in reference to the center of the operator’s screen. Because of practical difficulties in nonstandard alignment, some clinicians approximate the CSC’s position using the coaxially sighted corneal reflex. With this method, the patient looks directly at a luminous fixation point centered in the circular rings of the imaging device. The anterior corneal surface’s first Purkinje image, i.e., the reflection of the light on the exterior corneal surface, is used to approximate the location of the CSC. This approximation method reportedly locates a point on average 0.02 ± 0.17 mm (range: −0.43 mm to +0.68 mm) from the actual CSC in normal eyes; this, however, may not be the case in diseased or surgically altered corneas.
The third major and newest corneal reference point is called the thinnest corneal point (TCP), defined as the thinnest point or zone of the entire cornea. It is measured using various tomography instruments, which enable a mathematical three-dimensional reconstruction of the in vivo pachymetric distribution map, allowing one to evaluate the spatial variation of the thickness profile over the entire cornea. In the normal cornea, the average location and value of the TCP is 0.4-mm inferior and 0.4-mm temporal to CSC and 5-µm less thick than the central corneal thickness at the CSC.
Optical properties
Light refraction
The main optical measurements that determine the total refractive power of the eye are the anterior and posterior curvatures of both the central cornea and lens, the depth of the anterior chamber, and the axial length of the eye ( Fig. 4.4A ). Because this chapter is strictly about the cornea and sclera, we will focus only on the optical properties of the cornea. Refractive power and aberrations induced by the optics of the cornea are primarily due to corneal curvature and contour, respectively. Both are descriptors of corneal shape. The contour of the anterior corneal surface is basically of an aspheric geometry. The corneal apex defines the point of greatest refractive power or steepest curvature, and it then gradually and variably flattens from the apex to the periphery. Corneal asphericity has been known for over 100 years and has been modeled by various mathematical formulas in order to derive a quantitative approximation of contour. The central optical zone of the anterior corneal surface best corresponds to that of a conic section using the following formula, which requires knowing only two conic fit parameters— Q and R .
Q=p−1=(ba)2−1=Ra−1=(1−e2)−1

Q is a unitless asphericity factor or expression of the rate of curvature change from the apex of the cornea to the periphery ( Fig. 4.4B ); p is a geometric form factor; a and b are horizontal and vertical semimeridian hemi-axes; R is the apical radius of curvature; and e is the eccentricity. Q averages −0.4 in early childhood, but then gets gradually slightly less negative with age such that the central optical zone has a mean Q of −0.2 in adulthood (range: −0.81 to +0.47). Q < 0 describes a prolate contour where the rate of curvature change from the apex is less than that of a sphere ( Fig. 4.4C ); most normal corneas are prolate, which is advantageous in that it compensates for spherical aberrations induced by larger pupil sizes, which project misaligned peripheral rays of light on the fovea. Q = 0 describes a spherical contour where the rate of curvature change from the apex to the periphery is zero, and Q > 0 describes an oblate contour where the rate of curvature change from the apex is more than that of a sphere ( Fig. 4.4C ). Oblate contours are typically present in 20% or less of the normal population. Interestingly, asphericity can significantly change after surgery, especially excimer laser keratorefractive surgery, usually resulting in various oblate contours. Although the contour of the central optical zone of the anterior corneal surface is the most important for directly impacting foveal vision and on-axis aberrations (spherical aberrations, coma, and other HOAs), recent study has also shown that the peripheral optical zone is important for off-axis aberrations (e.g., glare, halos, starbursts). This peripheral optical zone does not fit a conic section well, but rather fits a ninth-order polynomial formula best and has a measured Q of −0.4 in adulthood when the central 10 mm of cornea is best fit to this formula. A few reports on the posterior corneal surface suggest it also has a prolate contour with a Q of −0.4, but its contribution to the total optical aberrations of the eye is less well known.
The actual total corneal dioptric power of the central 4.0 mm of cornea reportedly averages 42.4 ± 1.5 D (range: 38.4–46.3 D) of the eye’s total dioptric power of 60 D. The location of the corneal apex compared with the CSC (generally <1 mm from the CSC and on average 0.7 D steeper than that at the CSC), the degree of asphericity of the anterior corneal surface, and the degree of anterior corneal surface-to-posterior corneal surface ratios can vary widely from one individual to another or even change with age in an individual. For these reasons, it is difficult to take these general population-averaged results as empirically useful values. An individual’s total corneal power along the line of sight of the eye should probably best be measured using the Gaussian optics formula:
Ptotalcornea=nc−nairrant+na−ncrpost(dnc×(nc−nairrant)×(na−ncrpost))
Where P totalcornea equals diopters of optical power; n air , n c , and n a are the indices of refraction in air (1.000), cornea (1.376), and aqueous humor (1.336), respectively; r ant and r post are the radii of curvature of the anterior (0.0078) and posterior (0.0065) corneal surface in meters, respectively; and d is the central corneal thickness (0.00054) in meters.
42.18D=1.376−10.0078+1.336−1.3760.0065−(0.000541.376×(1.376−10.0078)×(1.336−1.3760.0065))
Therefore, the calculated total optical power of the cornea using known average major reference values is 48.21 − 6.15 + 0.12 = 42.18 D, which agrees closely with the actual mean of 42.4 D found in the study cited previously.
Because the cornea is thinner in the center than in the periphery, it should act as a minus lens, but functions as a plus lens because the aqueous humor neutralizes most of the minus optical power on the posterior corneal surface. If we compute the power of the posterior corneal surface in air, we find the following:
Ppostcornea=na−ncrpost=1−1.3760.0065=−57.85
The resulting calculated total optical power of the cornea would then be 48.21 − 57.85 + 1.12 = −8.52 D, which is a minus lens.
From the foregoing calculations, it is obvious that the most important refractive surface for humans is the anterior corneal surface. However, if a large air bubble is placed in the anterior chamber so that it contacts the corneal endothelium or if the anterior surface of the cornea is submerged in water, tremendous changes in the refractive power of the eye occur. For example, when the eye is open underwater during swimming, the optical imagery is extremely blurred; the index of refraction of water (1.333) is quite similar to that of the tear film and cornea (1.376). Thus, most of the optical power of the anterior corneal surface is lost. If the air-tear film interface is maintained by the use of a mask or goggles, then underwater vision is as sharp and clear as normal terrestrial vision. If the central optical zone of the anterior corneal surface is regular, yet not uniformly spherical in each meridian, the condition of astigmatism usually results. With astigmatism, a distant fixation point is refracted by the cornea and lens to become two focal lines rather than a sharp image point. However, if the central optical zone is irregular, then irregular astigmatism usually results. In adult humans, the conjunctival surface area has been measured at approximately 17.65 cm 2 and the corneal surface area measures 1.38 cm 2 , giving a conjunctival-to-corneal surface area ratio of 12.8, which is important for drug delivery calculations.
Light transmission
The cornea is an excellent example of the structural characteristics that a tissue needs to fulfill its dual role of transparency and mechanical support. Tissue transparency is rarely seen in the animal kingdom outside the eye. In fact, the only structures in humans where this property is seen are in the eye (e.g., cornea, lens). Corneal transparency has occupied scientists for over half a century and initial transparency theories focused on the extracellular stromal matrix while ignoring the cells of the stroma. Corneal transparency is now thought to be attributable to both the lattice-like arrangement of collagen fibrils in the corneal stroma and the transparency of cells that reside in the cornea. In summary, all currently viable transparency theories agree with these major points:
- 1.
Each corneal collagen fibril is an ineffective scatterer of light. Although inefficient, based on the large number of fibrils in the human corneal stroma, destructive interference of scattered light must occur due to the short-range order of collagen fibrils in the stroma.
- 2.
Each keratocyte nucleus mildly scatters some light. However, the cell body is an ineffective scatterer of light because of transparent intracellular cytoplasmic water-soluble corneal crystallins, its thinness, and because keratocytes are evenly distributed in the corneal stroma through a clockwise circular arrangement, so light transmission is hardly affected.
- 3.
Scattering of light is minimal in the cornea because it is thin.
- 4.
If an increased refractive index imbalance occurs between fibrils, keratocytes, or extrafibrillar matrix, light scattering can increase tremendously in the corneal stroma, resulting in loss of transparency.
To understand these theories and generalized principles, one needs to start with the structure of the corneal stroma.
The corneal stroma accounts for 90% of the corneal thickness. It is predominantly composed of water (3.5-gram H 2 O/gram dry weight) that is stabilized by an organized structural network of insoluble and soluble extracellular and cellular substances ( Table 4.1 ). The dry weight of the adult human corneal stroma is made up of collagen, keratocyte constituents, proteoglycans, corneal nerve constituents, glycoproteins, and salts ( Table 4.1 ). Overall, these corneal components work together to maintain and establish a transparent cornea. Although the cornea primarily absorbs most ultraviolet (UV) light, it transmits almost all visible (400–700 nm) and infrared (IR) light up to a wavelength of 2500 nm, with its peak transmission rate of 85% to 99% in the visible spectrum ( Fig. 4.5A ). The remaining portion (1%–15%) is scattered in all directions by the cornea in a wavelength-dependent fashion, with violet light being most affected. Clinical slit-lamp examination and in vivo confocal microscopy suggest that most of the light scatter is due to cellular components in the cornea rather than extracellular matrix. Relative amounts of light scattering owing to each stromal constituent are the following: endothelial cells > epithelial cells > nerve cells > keratocytes >> collagen fibrils or extracellular matrix ( Fig. 4.5B ). In fact, the in vivo confocal microscope shows that the highest area of the light scatter occurs where differences in the indices of refraction are high, such as at the air-tear film interface of the epithelium. Within the corneal stroma, light scatter predominantly comes from the stroma-plasma membrane interface of nerve cells and the cytoplasm-nuclear interface of keratocytes. With corneal edema or corneal scarring, loss of corneal transparency has been found to be primarily due to changes in the light-scattering characteristics of keratocytes rather than alterations in the extracellular matrix. In these conditions, keratocytes scatter considerably more light than normal corneas, particularly their cell bodies and dendritic processes ( Box 4.1 ).
Component | Wet weight (%) | Dry weight (%) |
---|---|---|
Cornea | ||
Water | 78 | – |
Matrix | 66 | |
Cellular | 12 | |
Collagen | 15 | 71 |
Proteoglycans | 1 | 9 |
Keratocytes | 1 | 10 |
Other | 5 | 10 |
Sclera | ||
Water | 68 | – |
Collagen | 27 | 77 |
Elastin | 1 | 2 |
Proteoglycans | 1 | 3 |
Fibrocytes | 1 | 3 |
Other | 2 | 6 |


Until recently, total corneal power was usually derived clinically from keratometric or topographical measurements of anterior corneal radius of curvature. The cornea was regarded as a single refractive surface with an effective refractive index of 1.3375, also known as the keratometric index of the cornea, because no accurate measurement of an individual’s posterior corneal radius of curvature could be measured clinically. Although not individualized for each patient, this mathematical approximation was used primarily for its convenience in the clinical setting; however, with the advent of clinical Scheimpflug tomography (e.g., Pentacam, Oculus Inc., Lynnwood, WA, USA) and other new tomography instruments soon to come on the market, the problems of accurately measuring an individual’s actual posterior corneal surface curvature and corneal thickness along the line of sight in the clinical setting have been predominantly resolved, although finding the exact location of the line of sight is still sometimes cumbersome, particularly in postrefractive surgery patients. Alternatively, total corneal power can still be approximated using these older devices, but at least now more accurately, since the keratometric index has been calculated to be around 1.328.
Collagen
Collagen is a structural protein organized into a relatively inextensible scaffold of water-insoluble fibrils that form the basic structural framework of a connective tissue. The corneal collagens are functionally important in establishing tissue transparency and in resisting tensile loads, ultimately defining the size and shape of the tissue. Collagen molecules measure 1.5 nm in width by 300 nm in length and are composed of a triple helix of three alpha chains. Of the 28 different types of collagen, currently 13 are known to occur in the human cornea. Upon secretion from the cell, the propeptide form of the collagen molecule is cleaved and the monomer of the collagen molecule is assembled into fibril-forming, nonfibril-forming, or fibril-associated collagens with interrupted terminals (FACIT) in a surface recess on the keratocyte or, sometimes, begins assembly inside the cell.
The most common collagen molecule in the cornea is type I (58%), which usually aggregates into structural, banded fibrils ( Fig. 4.6B ), as seen on transmission electron microscopy, by ordering into a quarter-staggered parallel arrangement that is further stabilized in position by covalent intramolecular and head-to-tail intermolecular immature divalent crosslinks in a posttranslational enzymatic step using lysyl oxidase ( Fig. 4.6A and 4.6C1–3 ). With increasing maturity, a spontaneous conversion to mature crosslinks occurs in which intramolecular, intermolecular, and interfibrillar mature trivalent crosslinks replace the immature divalent ones resulting in corneal collagen fibrils with improved mechanical properties ( Fig. 4.6C , middle diagram). Both immature and mature crosslinks occur between lysine and hydroxylysine side-chains. After maturation, the turnover or half-life of collagen molecules and fibrils becomes very slow; the concentration of mature crosslinks, however, remains stable, whereas high levels of random intramolecular, intermolecular, and interfibrillar nonenzymatic glycation crosslinks accumulate, predominantly between lysine and arginine residues ( Fig. 4.6C , bottom diagram). These nonenzymatic age- or diabetes-related glycation crosslinks initially enhance the mechanical properties of fibrils, resulting in stiffer, stronger, and tougher fibrils than normal, but occasionally they can go too far, making the tissue too brittle or inextensible to function normally. Type I fibrils are generally heterotypic (type I and type V [15%] collagen molecules) as they are composed of two or more types of collagen molecules ( Fig. 4.6B ). This may serve as a fine-tuning mechanism for controlling a fibril’s structural characteristics, such as fibril size and interfibrillar connectivity.

Type I fibrils usually reach certain specific diameters based on their composition and ratio of heterotypic collagen molecular types. They are restricted from further lateral accretion or growth and are permitted to fuse and grow axially due to interactions with small leucine-rich proteoglycans covalently bound to their external surface. Through surface interactions, they connect to various other fibril-forming, nonfibril-forming, or FACIT collagens, which overall link differrent levels of structural organization ( Fig. 4.6 ). Thus, the surface properties of type I fibrils are a major determinant of intrafibrillar and interfibrillar biomechanical properties of the tissue. The other major determinants are the direction of the fibrils and the suprafibrillar architectures of the tissue, which overall define the hierarchical structure of the tissue. They typically form uniform 25-nm diameter fibrils in the stroma proper with only slight variability. (Note: the diameters used in this chapter are based on transmission electron microscopic [TEM] data; x-ray scattering of ex vivo unprocessed human tissue suggests that a 24% to 36% shrinkage artifact occurs by fixing and processing tissue for TEM studies.) Bowman’s layer is the main exception as it has uniform 22-nm diameter type I fibrils, which are epithelial in origin as opposed to keratocyte in origin. The diameter of type I fibrils remains constant across most of the central cornea (mean: 25 ± 2 nm; range: 18–32 nm), but then gradually thickens another 4 nm at about 5.5 mm from the center of the cornea, eventually increasing to up to 50 nm at the limbus. Similarly, interfibrillar spacing between nearest neighbor type I fibrils remains constant in the central cornea (mean: 20 ± 5 nm; range: 5–35 nm), then gradually increases another 5 nm at about 4.5 to 5 mm from the center of the cornea before increasing even more rapidly up to the limbus. Type I fibril diameters and interfibrillar spaces do not seem to vary significantly with depth in the cornea. Although the refractive index of type I fibrils (1.47) is different from that of the extrafibrillar matrix (1.35), the highly uniform small size and highly uniform small interfibrillar spaces, along with the predominantly parallel directionality of these fibrils, results in a highly ordered lattice-like arrangement. This arrangement is not a true crystalline lattice, but more of a short-range order that allows transparency of the cornea owing to destructive interference ( Fig. 4.7 ).

Type VI collagen (24%) is the second most common type of collagen in the corneal stroma. It is present in an unusually high amount compared with most other connective tissues in the body, but it is also unique in that it is only able to aggregate into repeating tetramers of type VI molecules that are stabilized by disulfide crosslinks ( Fig. 4.8A ). Thus, it forms 10- to 15-nm diameter nonbanded filaments with 20- to 30-nm diameter beaded ovals having a periodicity of 100 nm. Functionally, type VI filaments act as a bridging structure in the interlamellar space, as they bind corneal lamellae together diffusely throughout the stroma where they directly cross one another ( Fig. 4.8B,C ). Along with type XII and XIV FACIT collagens, type VI collagen may bridge fibrils together in the interfibrillar space ( Fig. 4.8D ). Overall, this suprafibrillar architecture results in a one-dimensionally ordered Bowman’s layer and a three-dimensionally ordered stroma proper.

Although difficult to count precisely, the central corneal stroma reportedly consists of approximately 300 corneal lamellae, while the peripheral cornea consists of approximately 500. Although most corneal stromal lamellae extend from limbus to limbus and cross adjacent lamellae at various angles, randomly in the anterior stroma and nearly orthogonal to one another in the posterior two-thirds, various regional differences in lamellar size, directionality, and amount of interweaving are also found ( Figs. 4.9 and 4.10 ). The anterior third of the stroma proper has thinner (0.2- to 1.2-µm thick), narrower (0.5- to 30-µm wide), and mostly obliquely oriented lamellae (mean 18 ± 11 degrees [range 0–36 degrees]) with extensive vertical and horizontal interweaving ( Fig. 4.9A ). The posterior two-thirds has thicker (1- to 2.5-µm thick), wider (100- to 200-µm wide), and mostly parallel-oriented lamellae (mean 1 ± 2 degrees [range 0–5 degrees]) with only slight horizontal interweaving ( Figs. 4.9B and 4.10B,C ). In the most superficial layers of the stroma, the interwoven lamellae actually attach or possibly seem to originate from the posterior surface of Bowman’s layer in a polygonal fashion, creating an anterior corneal mosaic pattern ( Fig. 4.9A , inset) that can be seen on the anterior corneal surface under certain circumstances. The attached fibrils to Bowman’s layer seem to be homologous to sutural fibers in the shark cornea and embryologically may be remnants of the primary acellular stroma. Those attaching or originating fibril bundles are usually oriented obliquely to Bowman’s layer, but are sometimes noted to be almost perpendicular to it. Finally, excepting those fibrils and lamellae in the anterior-most region of the stroma that attach to Bowman’s layer, the remaining type I fibrils and corneal lamellae stretch across the cornea from limbus to limbus in a belt-like fashion, where they turn and form a circumferential annulus approximately 1.0- to 2.5-mm wide around the cornea. This annulus is thought to maintain the curvature of the cornea, while blending with limbal collagen fibrils.


Keratocytes
Keratocytes make up the second major component of the corneal stroma’s dry weight. They are sandwiched between collagenous lamellae, forming a closed, highly organized syncytium. They function as modified fibroblasts during neonatal life, forming most of the extracellular matrix of the stroma. Subsequently, they remain in the cellular stroma throughout life as modified fibrocytes, where they maintain the extracellular matrix of the corneal stroma. Keratocytes can become metabolically activated or fibroblastic again if the corneal stroma is wounded. The adult human corneal stroma has approximately 2.4 million keratocytes communicating with each other through gap junctions present on their long dendritic processes ( Figs. 4.11A ). In adulthood, keratocytes occupy 10% of the stromal volume, decreasing from 20% in infancy, and on two-dimensional cross-sectional views appear to be sparse, flattened, and quiescent (scant intracytoplasmic organelles) cells lying between corneal lamella ( Fig. 4.11B ). In actuality, keratocytes are three-dimensional stellate-shaped cells composed of a 15- to 20-µm diameter cell body with numerous dendritic processes that extend up to 50 µm from the cell body. Tangential sections of the normal cornea suggest that these cells more densely populate the stroma than originally thought and are more metabolically active in the resting state than initially presumed, as in tangential section, an abundance of cytoplasmic organelles are commonly seen ( Fig. 4.11C,D ).

Tangential sections show that the anterior stromal keratocytes contain twice the number of mitochondria as the posterior two-thirds of the stroma, which correlates with the higher oxygen tension of the anterior stroma. It has also been demonstrated that a higher stromal cell density occurs in the anterior stroma than in the mid- or posterior stroma, whereas a higher cell volume-to-extracellular matrix ratio occurs in the posterior stroma compared with the anterior- or midstroma. These views also show that in all levels the keratocytes are highly spatially ordered as they turn in a clockwise direction like a cork-screw. In vivo confocal microscopy of normal human corneas has shown stromal cell densities averaging around 20,000 keratocytes/mm 3 , with a focal zone of increased cell density directly under Bowman’s layer, averaging 35,000 keratocytes/mm 3 in the anterior-most layer that gradually tapers to 20,000 keratocytes/mm 3 over the initial 60 to 100 µm in depth. Confocal microscopy has also shown that stromal cell density decreases with age at a rate of approximately 0.5% per year of life with the anterior stroma declining 0.9% per year, midstroma 0.3% per year, and posterior stroma 0.3% per year.
Studies using immunohistochemistry or electron microscopy suggest that not all the cells in the corneal stroma are actually keratocytes, but some are one of three types of bone marrow-derived immune cells: “professional” dendritic cells, “nonprofessional” dendritic cells, and histiocytes. Recent studies also found evidence of a small resident subpopulation of adult stromal stem cells, also known as keratocyte progenitor cells/corneal stromal stem cells, primarily in the periphery of the corneal stroma near the limbus. The immune cells appear to play a pivotal role in the induction of immune tolerance versus immune initiation in cell-mediated immunity, and the stromal histiocytes have a role in innate immunity as phagocytic effector cells. The presence of adult stromal stem cells helps explain how the slow replacement and renewal of keratocytes occurs after injury, surgery (e.g., epikeratophakia or penetrating keratoplasty [PK]), or toxicity to the central corneal stroma (e.g., mitomycin C or refractive surgery) ( Box 4.2 ).
The principle of using contact lenses to correct refractive errors is that one essentially replaces the powerful anterior corneal refractive surface to that of the anterior contact lens surface ( Fig. 4.4A , right top diagram ). Upon application of a contact lens, the cornea’s anterior surface is rendered ineffective as it is bathed with aqueous tears and the air-contact lens interface now becomes the predominate refractive surface of the eye. Soft contact lenses are typically used to treat spherical and regular astigmatism and rarely cause any permanent pathologic changes or alterations to the cornea unless there is a complication, such as corneal infection, infiltrate, or toxic conjunctivitis.
The prevalence of complications in contact lens wearers is currently about 5% per year of wear. Soft contact lenses, however, have been noted to cause some acute physiologic changes to the cornea, including epithelial thinning, hypoesthesia, superficial punctate keratitis, epithelial abrasions, stromal edema, and endothelial blebs. They also cause chronic changes including corneal vascularization, stromal thinning, corneal shape alterations, and endothelial cell polymegathism and pleomorphism (signs of endothelial cell stress). These are all thought to result from the contact lens-induced hypoxia and/or hypercapnia of the tissue.
Most of these physiologic alterations, particularly the chronic ones, are markedly less common now with the introduction of daily-wear high oxygen transmissibility lenses, such as silicone hydrogel contact lenses. In contrast, hard contact lenses treat spherical, regular astigmatism, and even some cases of irregular astigmatism. Hard contact lenses cause the same acute and chronic physiologic changes to cornea as soft contact lenses, although they do more commonly cause corneal shape alterations to occur because they induce more mechanical pressure on the anterior corneal surface. In fact, this is the basis for the practice of deliberately fitting tight, overly flat rigid gas-permeable contact lenses with the aim of flattening the central cornea to reduce myopia in a technique known as orthokeratology. The fitting of contact lenses is highly empirical. Considerable trial and error can be involved in adjusting variables such as contact lens material, size, curvature, and other patient-related factors that are used to arrive at an appropriate correction and comfort level for each individual contact lens wearer.
Proteoglycans
Proteoglycans make up the third major component of the corneal stroma’s dry weight. They are water-soluble glycoproteins made up of a core protein with a covalently attached anionic polysaccharide side-chain called a glycosaminoglycan (GAG). The core proteins are noncovalently attached to collagen fibrils uniformly throughout the tissue, whereas the GAG side-chains extend into the interfibrillar space where they act as a pressure-exerting polyelectrolyte gel. The cornea collapses to approximately 20% of its original volume if the proteoglycans of the corneal stroma are precipitated out with cetylpyridinium. It has become apparent that the primary functions of proteoglycans are to provide tissue volume, maintain spatial order of collagen fibrils, resist compressive forces, and give viscoelastic properties to the tissue, as well as having a secondary role in regulating collagen fibril assembly. Corneal proteoglycans were previously referred to as extrafibrillar amorphous ground substance, as their water-soluble state made it difficult to fully delineate them with light and electron microscopy ( Fig. 4.12A ). It was not until an electron-dense cationic dye called cupromeronic blue and a critical electrolyte concentration of 0.1 M MgCl 2 were used in combination to specifically stain for the sulfate-ester groups on corneal proteoglycans that the shape, size, arrangement, and location of this material were observed with light and electron microscopy ( Fig. 4.12B ).

Since that discovery, it has become apparent that corneal proteoglycans are not amorphous, but rather tadpole-shaped molecules composed of a 10- to 15-nm diameter globular core protein with a covalent attached that is 7-nm wide × 45 to 70 nm in length. The GAG side-chain attaches to the covalent. They are arranged in the corneal stroma perpendicular to collagen fibrils with a constant spacing of around 65 nm between each other along the collagen fibrils. Their core proteins noncovalently bind to collagen fibrils in specific gap zones along the peripheral portions of the collagen fibril. The core proteins with dermatan sulfate side-chains bind to “d” and “e” gap zones and those with keratan sulfate side-chains bind to “a” and “c” gap zones. GAGs are highly negatively charged stiff polymers that extend into the interfibrillar space and form antiparallel duplexes with adjacent GAG side-chains ( Fig. 4.12C ), thereby linking different next-nearest-neighbor collagen fibrils together by forming dumbbell-like structures. The genes that produce the core proteins have been cloned, and four types of corneal stromal proteoglycan core proteins have been identified: decorin, lumican, keratocan, and mimecan. Decorin contains a single dermatan sulfate GAG side-chain ( Fig. 4.12D ), whereas lumican and mimecan have a single keratan sulfate GAG side-chain and keratocan has three keratan sulfate GAG side-chains ( Fig. 4.12D ). Thus, there are four known types of proteoglycan core proteins and only two types of GAGs, keratan sulfate (60%) and dermatan sulfate (40%), found in the human corneal stroma. GAGs are polymers of repeating disaccharide units of galactose and N-acetylglucosamine or iduronic acid and N-acetylgalactosamine, respectively.
Because the core protein tail and their associated GAG side-chains are post-translationally added to core proteins in the Golgi apparatus, there seems to be some flexibility in how long or how sulfated they can become depending on the function of the connective tissue producing them. The human cornea is unique in that the core protein tail and its associated GAG side-chains are fibril-associated and small in length (keratan sulfate ∼45 nm and dermatan sulfate ∼70 nm), with a higher amount over-sulfated compared with other connective tissues. A comparative study of corneas from 12 mammalian species suggests that dermatan sulfate is the preferred proteoglycan in oxygen-rich environments, such as in the thin cornea of mice, or is seen predominantly in the anterior portion of the thicker cornea of mammals, such as humans or rabbits. Keratan sulfate is a functional substitute produced through an alternate metabolic pathway in thicker corneas, especially in the posterior portion where oxygen levels may drop precipitously. Functionally, this duality is quite useful because dermatan sulfate appears to be more efficient at holding water; it absorbs less water than keratan sulfate, but holds most of it in a tightly bound, non-freezable state. This is consistent with the fact that dermatan sulfate is more abundant in the anterior corneal stroma in humans, which is the region of highest oxygen tension and most affected by evaporation. In contrast, keratan sulfate is more abundant in the posterior corneal stroma ( Fig. 4.13 ). This is the region of lowest oxygen tension, least affected by evaporation, and the area where the need for loosely bound water is required for transport across the endothelium via the metabolic pumps.

Corneal nerves
The epithelium of the cornea is the most richly innervated tissue of the body with about 16,000 nerve terminals/mm 2 (∼2.2 million nerve endings), about 300 to 400 times more dense than skin. Most of the nerve fibers in the cornea are sensory in origin, responding to mechanical, chemical, and temperature stimuli, and are derived from the ophthalmic branch of the trigeminal nerve (CN III 1 ) ( Fig. 4.14A ). Refer to Chapter 16 (Sensory Innervation of the Eye) for full details of the innervation pathway of the cornea. Although all mammalian species receive variable proportions of nerve fibers in the cornea from the sympathetic and parasympathetic autonomic nervous system, human corneas appear to be on the extreme end of this spectrum as their corneas have a very small proportion of their nerve fibers derived from the autonomic nervous system.

Electrophysiological studies have shown that the cornea’s receptive nerve field is composed primarily of polymodal nociceptors (70%) followed by mechanonociceptors (20%) and then cold-sensitive nociceptors (10%). Using in vivo laser confocal microscopes, one can only evaluate morphologically the corneal nerves from the main nerve trunks up to sub-basal plexus (SBP) as the resolution and contrast of these images is not capable of visualizing the final nerve branches and free nerve terminals in the corneal epithelium.
Because corneal nerve fibers ultimately terminate in the brainstem, it appears that interneuron intermediate pathways must relay the information to the sensation areas of cerebrum. Additionally, there must be intermediate relays to efferent systems that trigger the reflex pathways of involuntary blinking via orbicularis motor innervation from CN VII and reflex tearing via parasympathetic innervation of lacrimal gland. The intricate central nervous system (CNS) details of these specific pathways are currently unknown.
Corneal sensitivity is a valuable clinical measure of corneal health, as corneal nerves directly maintain the health of corneal epithelium through direct trophic factors. Corneal sensitivity also serves a protective role in warning the host of possible dangers to the normal healthy state and in maintaining an adequate basal tear secretion rate. It is usually tested clinically in a semiquantitative fashion with a Cochet and Bonnet esthesiometer, which is a thin (0.12-mm diameter) flexible nylon filament of variable length (0–6 cm). When the filament is long, it applies very little pressure to the corneal surface because it bends easily, whereas when short it applies a proportionally higher pressure before bending. The length is converted into pressure using a conversion table with a range of touch pressures between 11 and 200 mg/mm 2 . Corneal sensitivity is defined as the reciprocal of corneal touch threshold. It can be evaluated subjectively by asking patients when they feel touch upon the cornea, or objectively when a reflexive blink response is triggered. Refer to Chapter 16 for full details on the subjective and objective threshold in normal healthy corneas and those with disease or after surgery.
Corneal sensation also variably decreases with corneal disease (e.g., herpes simplex keratitis, diabetes, corneal dystrophies, keratoconus); following surgical procedures on the anterior segment of the eye and sometimes the posterior segment (e.g., panretinal photocoagulation); after application of certain topical medications (e.g., anesthetics, nonsteroidal anti-inflammatory drugs); and even after contact lens wear. As nerve regeneration occurs at the rate of approximately 1 mm per month, it may take up to 3 to 12 months or longer for corneal reinnervation and sensation to maximally recover, depending on the type and degree of surgical injury. After maximal recovery, the sensitivity in the portion of the cornea involved by the procedure often is less than that present prior to surgery, which means there is a potential for neurotrophic keratitis.
It has been discovered recently that after corneal nerve injury, microneuromas can develop during the regeneration process. These microneuromas, as well as injured corneal nerves themselves, exhibit altered functional properties where responsiveness to normal stimuli is impaired, yet paradoxically display abnormal intrinsic electrical excitability (i.e., spontaneous impulses or even abnormal responsiveness to normally minimal stimuli). Overall, this may produce hyperalgesia and/or dysesthesia, which may continue long term after surgery despite some attenuation. These neuropathic pain impulses are often perceived by the patient as dryness, foreign body sensation, and irritation after surgery, yet are not due to actual dryness or irritation of the cornea. These undesired, unpleasant sensations may best respond to ion channel antagonists rather than dry eye lubricating medications.
The mechanisms by which corneal nerves maintain the ocular surface and promote healing after eye injuries are currently under active research in several laboratories. Corneal nerves secrete neuropeptides, such as substance P and calcitonin gene-related protein, and neurotransmitters, such as acetylcholine, vasoactive intestinal polypeptide, and neurotensin, which are believed to be important in corneal epithelial function and proliferation.
Corneal stromal wound healing
The first published report specifically addressing the cellular reactions in the corneal stroma after injury appeared in 1958. It described the morphologic changes of stromal cells after different types of trauma and found that stromal cells lose their interconnecting dendritic processes immediately after injury, with many cells subsequently developing signs of degeneration. That report also described the appearance of morphologically unique, spindle-shaped corneal fibroblastic cells invading into the wound region during later stages of stromal healing. Since that time, many excellent animal-model studies of corneal wound healing have further addressed the changes in the extracellular matrix and the stromal cells after stromal injury. They suggest that corneal stromal injury is immediately followed by keratocyte apoptosis in the zone around the site of stromal injury, with a subsequent influx of transient mixed acute and chronic inflammatory cells; proliferation and migration of surviving keratocytes; and, finally, differentiation of the keratocytes into transiently metabolically activated cell types called activated keratocytes. This latter cell type is functionally important because it synthesizes and deposits the extracellular matrix of the stromal scar, while also degrading and remodeling the damaged cellular and extracellular tissues around the wound. Epithelial injury alone can also cause transient cellular injury to the underlying stroma, presumably from exposure of stroma to tear-related factors, resulting in apoptosis, proliferation, and differentiation into migratory keratocytes, as well as resulting in some anterior stromal edema. However, it does not appear to cause differentiation into activated keratocytes, hypercellularity, differentiation into myofibroblasts, or the stimulation of extracellular matrix production—all of which are seen with corneal stromal injury, whether by incision or excision. Myofibroblasts are characterized by the intracellular cytoplasmic appearance of α-smooth muscle actin, which helps impart contractile properties to the cell.
A number of studies have looked into the expression of cytokines and growth factors in normal and injured corneas. These studies tried to assess the relative importance of each specific factor in cornea wound healing, as it was known clinically and experimentally that epithelial-stromal interactions increase the number of proliferative and migratory keratocytes within the stromal wound compared with deeper corneal stromal injury, some of which differentiated beyond the activated keratocyte stage into myofibroblasts. Some studies focused even more specifically on strictly epithelial or tear-related cytokines or growth factors, as the epithelium and aqueous tears were found to be a major source of cytokines and growth factors. The major cytokines and growth factors studied to date include epithelial growth factor (EGF), fibroblast growth factor (FGF), interleukin-1, nerve growth factor (NGF), transforming growth factor beta (TGF-β), insulin, retinol, and Lysophosphatidic acid (LPA). TGF-β is currently thought to be the most important growth factor of this group with regard to stimulating a fibrotic reparative stromal scar phenotype. A recent study, however, has shown that local cytokine and growth factor-related influence on normal corneal stromal wound repair is predominantly an early wound-healing phenomenon, as cell-matrix interactions seem to take over in the later stages of wound healing. For example, integrity or return of a complete epithelial cell basement membrane after stromal injury seems to regulate epithelial-stromal interactions long term, as it decreases the production and, more importantly, the release of TGF-β from epithelial cells into the stroma. One gap in this area of research is how mechanotransduction pathways (mechanical load-induced intracellular signals) fit into this scheme, particularly in maintaining myofibroblast differentiation long term and thus corneal haze. Other cytokines and growth factors of this group were also found to have some minor complementary or even competing roles with TGF-β, and in other cases they had no role at all in corneal wound healing. For example, NGF is complementary to TGF-β, as it too is known to stimulate myofibroblastic cellular transformation independent of TGF-β, but it does so without enhancing keratocyte proliferation or extracellular matrix deposition. In another example, all- trans retinol (vitamin A), which is reflexively secreted in the aqueous tears from its storage location in the lacrimal gland, has a completely different role than wound healing, as it maintains the moist, mucosal ocular surface phenotype via gene transcription regulation; thus, it ultimately controls the rate of ocular surface cell proliferation and differentiation (i.e., prevents keratinization and squamous metaplasia). Once activated, keratocytes exhibit a wide range of cellular responses, including increased tritiated thymidine uptake (indicating increased proliferation); initiation of protease and collagenase activity; phagocytosis; interferon, prostaglandin, and complement factor 1 production; and fibronectin, collagen, and proteoglycan secretion.
Human studies of stromal wound healing concur with animal studies on most issues, with the following notable differences: adult human corneas heal less aggressively, more slowly, and not as completely as animal corneas. However, both animal and human studies show that corneal stromal wounds heal in two distinct phases: (1) an active phase, which results in the production of a stromal scar over the first 6 months after injury in humans, and (2) a remodeling phase, which improves corneal transparency and increases wound strength. This second phase occurs up to 3 to 4 years after injury in humans. Overall, the long-term result in human corneas is the production of a hypercellular fibrotic stromal scar in wound regions where epithelial-stromal interactions occur and a hypocellular primitive stromal scar in wound regions where keratocyte injury pathways occur. These two histologic wound types have functional differences, as the hypercellular fibrotic stromal scar is strong, but can look clinically hazy because of myofibroblastic cells populating this scar type. In contrast, the hypocellular primitive stromal scar is transparent, but it is very weak in tensile and cohesive strength and serves as a potential space for fluid, inflammatory cells, and microbes. An additional variable to consider in this scheme is that more precisely realigned wounds, such as sutured and unsutured wounds with minimal gaping and no epithelial cell plugging, heal better than poorly aligned wounds, such as wounds with wide wound gaping, epithelial plugging, or incarceration of Bowman’s layer, Descemet’s membrane, or uvea.
Barrier properties
Low-permeability barrier: the corneal epithelium
The external surface of the human cornea is covered by a stratified squamous epithelium (see Fig. 4.1D ). Unlike other epithelial surfaces, the corneal epithelium is specialized to exist over a moist, transparent, refractive avascular surface, and thus it is smooth and nonkeratinized. The corneal epithelium is composed of 4 to 6 cell layers about 50 µm in total thickness across the entire anterior corneal surface ( Fig. 4.16 ). It is continuous with the epithelium of the limbus (see Fig. 4.3B ). The basal epithelial cells actively secrete a basement membrane, around 90-nm thick at birth, composed of type IV collagen fibrils, laminin, heparan sulfate, and fibronectin. The corneal epithelial basement membrane, or basal lamina, increases in thickness with age, measuring around 300-nm thick in late adulthood. By electron microscopy, the basal lamina appears to be composed of two distinct layers: a 20- to 30-nm thick more anterior lamina lucida and a 30- to 60-nm thick more posterior lamina densa. Its function is similar to most basement membranes in that it serves as a scaffold for epithelial cell movement and attachment.

The cytoplasm of all epithelial cells contains mainly cytoskeletal intermediate filaments and has sparse cytoplasmic organelles. This aids in maintaining transparency. The predominant cytoplasmic protein is keratin, and actin filaments and microtubules are the other major ones. The basal cell layer stores large glycogen granules as a source of metabolic energy for times of stress during hypoxia or wound healing. The epithelial cells are held together by desmosomes, while the basal surface of the epithelium adheres to the basal lamina and underlying Bowman’s layer through an anchoring complex composed of hemidesmosomes, type VII collagen anchoring fibrils, and anchoring plaques ( Fig. 4.17 ). The epithelial cells differentiate from the basal layer to form one to three midepithelial layers of wing cells and finally to form one to two superficial squamous cell layers ( Fig. 4.16 ). The most superficial squamous cells at the external surface form a high-resistance (8–16 kΩ·cm 2 ) barrier to the external environment because they are all surrounded by a continuous band of apical zonula occludens tight junctions at their peripheral intercellular margins ( Fig. 4.18 ).

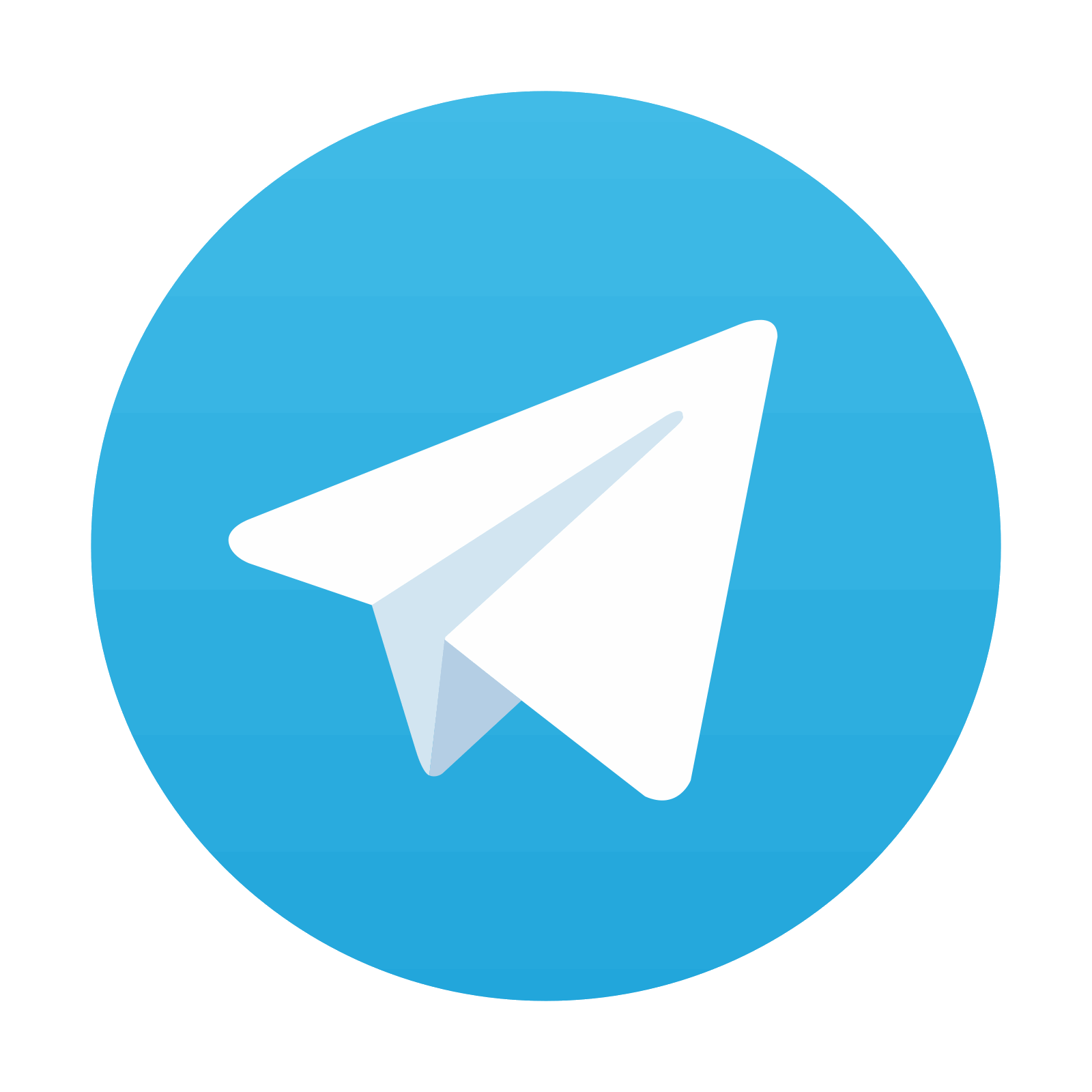
Stay updated, free articles. Join our Telegram channel
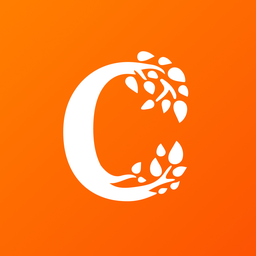
Full access? Get Clinical Tree
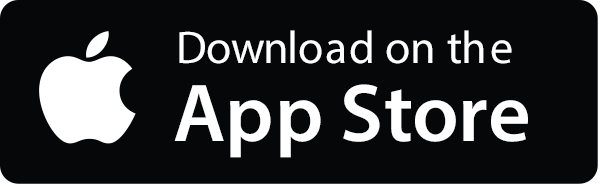
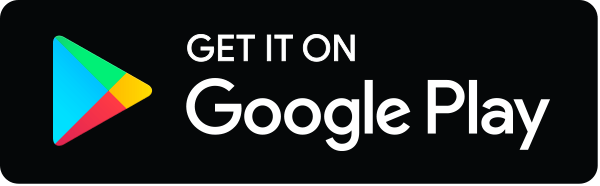
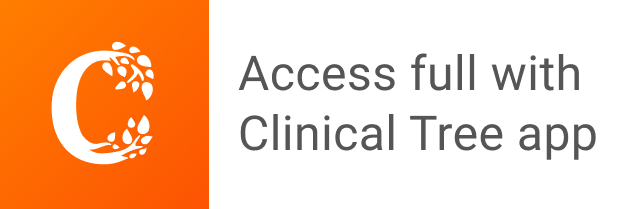