Introduction
The outermost, fibrous tunic of the human eye is the cornea and the sclera ( Fig. 4.1A,B ). Both are soft connective tissues designed to provide structural integrity of the globe and to protect the inner components of the eye from physical injury. The clear, transparent cornea ( Fig. 4.1A,C ) covers the anterior 1/6th of the total surface area of the globe, while the white, opaque sclera ( Fig. 4.1A ) covers the remaining 5/6ths. The cornea and the lens are the eye’s primary refractive structures and both have two key optical properties to this end – refractive power (light refraction) and transparency (light transmission). The presence of a healthy cornea is essential for good vision as it is basically the window of the eye. The cornea is most analogous to the external lens of a compound lens camera. By comparison, the sclera predominantly serves more of a biomechanical function and is analogous to the housing of the camera and lens.

The cornea is 540 to 700 µm thick and is arranged in five basic layers – epithelium, Bowman’s layer, stroma proper, Descemet’s membrane, and endothelium ( Fig. 4.1D ); each having distinctly different structural and functional charactistics. It also is composed of three major cell types – epithelial, stromal keratocyte, and endothelial cells. Two of these, epithelium and endothelium, form cellular barrier layers to the stroma. Thus, their resistance to diffusion of solutes and bulk fluid flow are of considerable importance to maintaining normal corneal function (resistance to diffusion of solutes and fluid flow: epithelium [2000] ≫ endothelium [10] > stroma [1]). All 3 can replicate through mitosis, but vary considerably in their in vivo proliferative capacity with epithelial cells having the highest rates of cell division and endothelial cells being the least renewable. This fact is seen clinically since epithelial cells can completely regenerate after injury (e.g. corneal abrasions), while endothelial cells, as a result of limited in vivo proliferation, are most commonly involved in age-related (e.g. Fuchs’ dystrophy) or injury-related disease (e.g. pseudophakic bullous keratopathy – PBK) ultimately resulting in corneal edema and bullous keratopathy. Corneal stromal keratocytes are a middle ground compromise between these two extremes. One major disadvantage of the epithelium’s high proliferative potential is that it can occasionally go unchecked, resulting in cancer (e.g. squamous cell cancers of the cornea), whereas keratocytes and endothelial cells do not have this risk.
The sclera is 0.3 to 1.35 mm thick and is arranged into three layers – episclera, scleral stroma proper, and lamina fusca; each having distinctly different structural and functional characteristics. The sclera is composed of only one major cell type, the sclera fibrocyte, which has moderate proliferative potential, like that of the corneal stromal keratoctye. As the sclera has no cellular barrier layers, its permeability properties are quite similar to that of the corneal stroma. The sclera is an excellent example of a tissue made for biomechanical stability as it is stiff, strong, and tough. As such, disease of the sclera commonly results in loss of tectonic support, abnormal size due to dysregulated growth as well as inflammatory conditions that commonly also affect the joints in the body. Many animals have a very rigid sclera that is often supported by bone or cartilage. Humans deviate from this extreme in that their sclera is a less rigid fibrous connective tissue, perhaps reflecting its need to maintain an even blood flow to the choroid and retina during large excursions in eye motility.
This chapter reviews and explains the structure and function of the cornea and sclera, providing a framework for understanding normal health and disease of each tissue with an emphasis on function.
Cornea
Embryology, growth, development, and aging
The foundations of contemporary corneal embryology and development stem from pioneering embryonic chick studies. Since few such detailed studies have been carried out in primates or other mammals, the information that follows has potential gaps in knowledge since species-related differences may exist. Following lens vesicle formation between 4 to 5 weeks’ gestation (27–36 days), surface ectodermal cells cover the defect left by lens vesicle invagination and become the primitive, undifferentiated corneal epithelium, composed initially of two cell layers. Similar to the avian cornea, the primitive corneal epithelium of primates and higher mammals immediately produces a primary acellular corneal stroma, or post-epithelial layer. In primates, this is seen as the gradual subepithelial addition of diagonal and then randomly oriented fibrillar elements, which later thicken into collagen fibrils that are slightly smaller in diameter than stromal collagen fibrils. The Bowman’s layer is thought to be a distinct, dense anterior-most remnant of this embryologic layer, which is first detectable by light microscopy around 20 weeks’ gestation. In contrast to humans, lower mammals, such as rabbits and rodents, have an underdeveloped primary acellular corneal stroma and its residual remnant, the Bowman’s layer, is indistinct and rather thin. Around 12 weeks’ gestation, a time period between eyelid fusion at 8 weeks’ gestation and eyelid opening at 26 weeks’ gestation, the epithelium differentiates to become a stratified, squamous epithelium 4 cell layers thick, which then produces an epithelial basement membrane. It remains 4 cell layers thick until approximately 6 months after birth when it reaches adult levels of 4–6 cell layers thick. Early in gestation, the epithelial basement membrane and anchoring complexes on the basal surface of the epithelium are absent. Rudimentary epithelial basement membrane and anchoring complexes only become detectable by 17 weeks of gestation. With further development in utero, the thickness and number of these structures gradually increases.
A first wave of neural crest-derived mesenchymal cells begins to extend beneath the corneal epithelial cells from the limbus around 5 weeks’ gestation (33 days); these cells form the primitive endothelium. The primitive endothelium is initially composed of two-cell layers. By 8 weeks’ gestation, it becomes a monolayer that starts to produce Descemet’s membrane, which becomes recognizable on light microscopy at 3–4 months’ gestation. The epithelium and endothelium remain closely opposed until 7 weeks’ gestation (49 days), when a second wave of mesenchymal cells begins to migrate centrally from the limbus between the epithelium and endothelium invading below and into most of the primary acellular stroma. The cells do not enter the anterior-most 10 µm of stroma, which lacks keratocan, a proteoglycan core protein signal thought to be required for cellular invasion. This second wave of cells forms the stroma proper, or secondary cellular corneal stroma, as production of lamellar collagen begins within a few days in a posterior-to-anterior fashion. It is believed that the invading mesenchymal cells, destined to become keratocytes, use the primary acellular stroma as a scaffold, primarily in the anterior third of stroma proper. This concurs with the significant lamellar interweaving and oblique lamellar orientation in the anterior third of post-natal human corneal stroma as well as the fact that each successive lamellar layer is rotated 1–2 degrees clockwise. This directional rotation is the same in both right and left eyes. In the posterior two-thirds of the stroma, the corneal stroma is composed of essentially orthogonal lamellae. By 3 months’ gestation, corneal nerves invade the stroma and eventually penetrate through the Bowman’s layer so that nerve endings develop in the epithelium. Studies also suggest that by 5 months’ gestation, tight junctions form around all the corneal endothelial cells and, by 5 to 7 months’ gestation, the in utero cornea becomes transparent as the density of functioning endothelial Na + /K + -ATPase metabolic pump sites increase to adult levels. By 7 months’ gestation, the cornea resembles that of the adult in most structural characteristics other than size. At birth in the full-term infant, the horizontal corneal diameter is about 9.8 mm and the corneal surface area is around 102 mm 2 . The cornea of the newborn infant is approximately 75–80 percent of the size of the adult human cornea ( Fig. 4.2A,B,C ), while the posterior segment is < 50 percent of adult size ( Fig. 4.2D ). At birth, the cross-sectional thickness of the 4-cell layer epithelium averages 50 µm, the Bowman’s layer averages 10 µm, the central stroma proper averages 500 µm, Descemet’s membrane averages 4 µm, and the endothelium averages 6 µm thick (total mean central corneal thickness ∼ 570 µm).

During infancy, the cornea continues to grow, reaching adult size around 2 years of age with a horizontal diameter of 11.7 mm, surface area of 138 mm 2 , anterior surface curvature of 44.1 D ( Fig. 4.2A ), and mean central corneal thickness of 544 µm ( Fig. 4.2C ). Thereafter, it changes very little in size, shape, transparency, or curvature, although a shift from with-the-rule to against-the-rule astigmatism has been associated with aging ( Fig. 4.2B ). Post-natal aging is associated with several structural changes to the corneal tissue including: (1) epithelial basement membrane growth or thickening of an additional 100 to 300 nm or a rate of approximately 30 nm per decade of life; (2) decreased keratocyte, sub-basal nerve fiber, and endothelial cell density, presumably from stress-induced premature senescence; (3) increased stiffness, strength, and toughness of the stroma, from enzymatic maturation- and non-enzymatic age-related glycation-induced cross-linking of collagen fibrils; (4) Descemet’s membrane thickening of an additional 6–11 µm or a rate of approximately 1 µm per decade of life; and (5) possible degeneration of extracellular matrix structures. These structural and cellular changes, however, minimally affect the optical and barrier functions of the cornea and perhaps improve the mechanical function. For example, corneal ectasia from natural causes, like keratoconus, is rarely seen after 40 years of age. Only three documented negative functional consequences are associated with aging – impaired corneal wound healing, decreased corneal sensation, and decreased extensibility of its tissue. In elderly individuals or younger individuals with lipid abnormalities, the cornea often becomes yellowish in the periphery of the cornea due to a fine deposition of lipid. This condition is called arcus senilis.
Major corneal reference points and measurements
When viewed anteriorly in the living eye, the adult human cornea appears elliptical ( Fig. 4.3A ) as the largest diameter is typically in the horizontal meridian (mean 11.7 mm) and the smallest is in the vertical meridian (mean 10.6 mm). This elliptical configuration is brought about by anterior extension of the opaque sclera superiorly and inferiorly. When viewed from the posterior surface, the cornea is actually circular ( Fig. 4.3A ), with an average horizontal and vertical diameter of 11.7 mm. The average radius of curvature of the anterior and posterior corneal surface is 7.8 mm and 6.5 mm, respectively, which is significantly less than the 11.5 mm average radius of curvature of the sclera. This results in a small 1.5–2 mm transition zone that forms an external and internal surface groove, or scleral sulcus, where the steeper cornea meets the flatter sclera ( Fig. 4.3A ). This sulcus typically is not obvious clinically because it is filled in by overlying episclera and conjunctiva externally. The tissue in this transition zone is known as the limbus ( Fig. 4.3B ), which averages 1.5 mm wide in the horizontal meridian and 2 mm wide in the vertical meridian. It is important because it contains adult corneal stem cell populations, contains the trabecular meshwork, which is the conventional outflow pathway for the aqueous humor, and is the inciting site of pathology in a few immunologic diseases. The limbus is also a major anatomic reference point for planning surgical entry into the anterior segment because it appears clinically as a blue transition zone. Therefore, an incision placed anterior to the blue zone is anatomically in the peripheral cornea, safely inside the trabecular meshwork and stem cells. The cornea is thinner in the center, measuring on average 544 ± 34 µm (range: 440–650 µm) with ultrasound pachymetry, and increases in thickness in the periphery to approximately 700 µm as it reaches the limbus. A meta-analysis of all cross-sectional and longitudinal corneal thickness studies over a 30-year time period showed that no significant age-related change in central corneal thickness occurred beyond the infant years.

The central cornea overlying the entrance pupil, which is a virtual image of the real pupil and is typically located 0.5 mm anterior to and 14 percent larger than the real pupil, contains the central or effective optical zone of the cornea ( Fig. 4.3C ). The central optical zone is the portion of the cornea that can successfully refract a cone-like bundle of light from a distant or near fixation target through the pupil of the eye and then directly refracts it onto the fovea. The central location and size of this central optical zone dynamically changes according to the location of the fixation target in relation to the cornea (e.g. more distant fixation target = larger-diameter central optical zone, off-center fixation target = off-center central optical zone) and the aperture of the real pupil in various lighting conditions since the bundle of light has the same cross-sectional shape (e.g. an oval pupil results in an oval central optical zone) as the pupil and its diameter are defined by the pupil’s diameter as well as the location of fixation target. The central optical zone’s diameter typically averages 3.6 ± 0.8 mm in photopic lighting and 5.8 ± 0.9 mm in scotopic light with a range between 1.5 to 9.0 mm depending on the lighting condition. The remaining cornea peripheral to this central optical zone is the peripheral optical zone, which can refract light through the entrance pupil, but it does so at such an acute angle that it only affects the more peripheral aspects of the retina including the macula ( Fig. 4.3C ); it rarely directly impacts foveal vision.
Within the central optical zone are three major corneal reference points ( Fig. 4.3D ) that are extremely useful in determining the shape, refractive power, and biomechanical properties of the cornea, since they are statically fixed reference points. The first is called the corneal apex; it is defined as the steepest point or area of the cornea. It typically is measured using a corneal keratographer, topographer, or tomographer and thus its exact location on the cornea is referenced in relation to the corneal intercept of the imaging device’s optical axis ( Fig. 4.3E ). The corneal intercept of the imaging device’s optical axis is termed the device’s axis point (DAP). On average, the corneal apex usually is located 0.8 mm temporal and 0.2 mm superior to the DAP or 0.5 mm temporal and 0.5 mm superior to the corneal intercept of the line of sight of the eye ( Fig. 4.3D ), but considerable inter-individual variability is found in the location of the corneal apex in comparison to the average. The clinical utility of the corneal apex is that it is of paramount importance in the selection and fitting of contact lenses and in determining the geometric aspheric shape of the cornea. Significant decentration of the corneal apex from the DAP may give a false interpretation of corneal shape (e.g. asymmetric shape). The easiest way to clarify whether this is real or due to artifact is to aim the imaging device’s optical axis directly at the corneal apex, which is more cumbersome and difficult to do than standard alignment position.
The second major corneal reference point is the corneal intercept of the line of sight, also known as the corneal sighting center (CSC). The line of sight is an actual principal axis of the real eye as opposed to a theoretical construct of a schematic eye (e.g. visual axis) and it is defined as the principal axis joining a distant fixation point to the fovea. It theoretically is always thought to cross the center of the entrance pupil, which may not be true in the real eye since it is known that the center of the entrance pupil dynamically and unpredictably shifts up to 0.7 mm in direction with changes in pupil diameter, whereas the line of sight is a statically fixed axis line. Thus, it perhaps is best thought of as a line connecting the fixation target to the CSC on the anterior surface of the cornea and then via an unknown non-linear pathway is refracted in the cornea and by the lens to focus on the fovea. The location of the line of sight and CSC are of utmost importance to know in certain clinical situations for getting the best visual results with keratorefractive surgery, particularly with retreatments and customized ablations, and for calculating the proper posterior chamber intraocular lens (PCIOL) power to put in during cataract extraction (CE), especially after previous refractive surgery or with anterior surface irregularities, since subclinical decentrations ≥ 0.5 mm or torsional misalignments ≥ 15° can yield unwanted visual symptoms (e.g. coma or other higher-order aberrations [HOAs]). On average, the CSC is 0.4 mm nasal and 0.3 mm superior to the dynamically, unfixed pupillary axis or 0.5 mm inferior and 0.5 mm nasal to the static, fixed corneal apex reference point ( Fig. 4.3D ), but its location overall is highly variable between different individuals. Using a keratographer, topographer, or tomographer in non-standard alignment position, the CSC can be directly determined by having the patient directly look at a luminous fixation point centered in the circular rings of the imaging device and then it is marked in reference to the center of the operator’s screen. Because of practical difficulties in non-standard alignment, some clinicians approximate the CSC’s position using the coaxially sighted corneal reflex, where the patient looks directly at a luminous fixation point centered in the circular rings of the imaging device and the anterior corneal surface’s first Purkinje image is used to approximate the location of the CSC. This approximation method reportedly locates a point on average 0.02 ± 0.17 mm (range: −0.43 mm to +0.68 mm) from the actual CSC in normal eyes; this, however, may not be the case in diseased or surgically altered corneas.
The third major and newest corneal reference point is called the thinnest corneal point (TCP), defined as the thinnest point or zone of the entire cornea. It is measured using various tomography instruments, which enable a mathematical 3D reconstruction of the in vivo pachymetric distribution map, allowing one to evaluate the spatial variation of the thickness profile over the entire cornea. In the normal cornea, the average location and value of the TCP is 0.4 mm inferior and 0.4 mm temporal to CSC and 5 µm less thicker than the central corneal thickness at the CSC. The location and value of TCP is important because it allows clinical differentiation of corneas with normal biomechanical properties from those with current or previous keratectasia since no normal cornea was found in a group with a TCP more than 1 mm distance from the CSC and less than 500 µm of thickness.
If the central optical zone of the anterior corneal surface is regular, yet not uniformly spherical in each meridian, the condition of astigmatism usually results. With astigmatism, a distant fixation point is refracted by the cornea and lens to become two focal lines rather than a sharp image point. On the other hand, if the central optical zone is irregular, then irregular astigmatism usually results. In adult humans, the conjunctival surface area has been measured at approximately 17.65 cm 2 and the corneal surface area measures 1.38 cm 2 , giving a conjunctival-to-corneal surface area ratio of 12.8, which is important for drug delivery calculations.
Optical properties
Light refraction
The main optical measurements that determine the total refractive power of the eye are the anterior and posterior curvatures of both the central cornea and lens, the depth of the anterior chamber, and the axial length of the eye ( Fig. 4.4A ). As this chapter is strictly about the cornea and sclera, we will focus only on the optical properties of the cornea. Refractive power and aberrations induced by the optics of the cornea are primarily due to corneal curvature and contour, respectively. Both are descriptors of corneal shape. The contour of the anterior corneal surface is basically of an aspheric geometry with the corneal apex defining the point of greatest refractive power or steepest curvature and then it gradually and variably flattens from the apex to the periphery. Corneal asphericity has been known for over 100 years and has been modeled by various mathematical formulas in order to derive a quantitative approximation of contour. The central optical zone of the anterior corneal surface best corresponds to that of a conic section using the following formula, which requires only knowing two conic fit parameters – Q and R :
Q = p − 1 = ( b a ) 2 − 1 = R a − 1 = ( 1 − e 2 ) − 1

Q is a unitless asphericity factor or expression of the rate of curvature change from the apex of the cornea to the periphery ( Fig. 4.4B ); p is a geometric form factor; a and b are horizontal and vertical semi-meridian hemi-axes; R is the apical radius of curvature; and e is the eccentricity. Q averages −0.4 in early childhood, but then gets gradually slightly less negative with age such that the central optical zone has a mean Q of −0.2 in adulthood (range: −0.81 to +0.47). Q < 0 describes a prolate contour where the rate of curvature change from the apex is less than that of a sphere ( Fig. 4.4C ); most normal corneas are prolate as it is advantageous in that it compensates for spherical aberrations induced by larger pupil sizes, which project misaligned peripheral rays of light on the fovea. Q = 0 describes a spherical contour where the rate of curvature change from the apex to the periphery is zero, while Q > 0 describes an oblate contour where the rate of curvature change from the apex is more than that of a sphere ( Fig. 4.4C ). Oblate contours are typically present in ≤ 20 percent of the normal population. Interestingly, asphericity can significantly change after surgery, especially excimer laser keratorefractive surgery, usually resulting in various oblate contours. Although the contour of the central optical zone of the anterior corneal surface is the most important for directly impacting foveal vision and on-axis aberrations (spherical aberrations, coma, and other HOAs), recent study has also shown that the peripheral optical zone is important for off-axis aberrations (e.g. glare, halos, starbursts). This peripheral optical zone does not fit a conic section well, but rather fits a ninth-order polynomial formula best and has a measured Q of −0.4 in adulthood when the central 10 mm of cornea is best fit to this formula. A few reports on the posterior corneal surface suggest it also has a prolate contour too with a Q of −0.4, but its contribution to the total optical aberrations of the eye are less well known.
The actual total corneal dioptric power of the central 4.0 mm of cornea reportedly averages 42.4 ± 1.5 D (range: 38.4–46.3) of the eye’s total dioptric power of 60 D. The location of the corneal apex compared to the CSC (generally less than 1 mm from the CSC and on average 0.7 D steeper than that at the CSC), the degree of asphericity of the anterior corneal surface, and the degree of anterior corneal surface-to-posterior corneal surface ratios can vary widely from one individual to another or even change with age in an individual. For these reasons, it is difficult to take these general population-averaged results as an empirically useful value. An individual’s total corneal power along the line of sight of the eye should probably best be measured using the Gaussian optics formula:
P t o t a l c o r n e a = n c − n a i r r a n t + n a − n c r p o s t − ( d n c × ( n c − n a i r r a n t ) × ( n a − n c r p o s t ) )
Where P totalcornea equals diopters of optical power; n air , n c , and n a are the indices of refraction in air (1.000), cornea (1.376), and aqueous humor (1.336), respectively; r ant and r post are the radii of curvature of the anterior (0.0078) and posterior (0.0065) corneal surface in meters, respectively; and d is the central corneal thickness (0.00054) in meters.
42.18 D = 1.376 − 1 0.0078 + 1.336 − 1.376 0.0065 − ( 0.00054 1.376 × ( 1.376 − 1 0.0078 ) × ( 1.336 − 1.376 0.0065 ) )
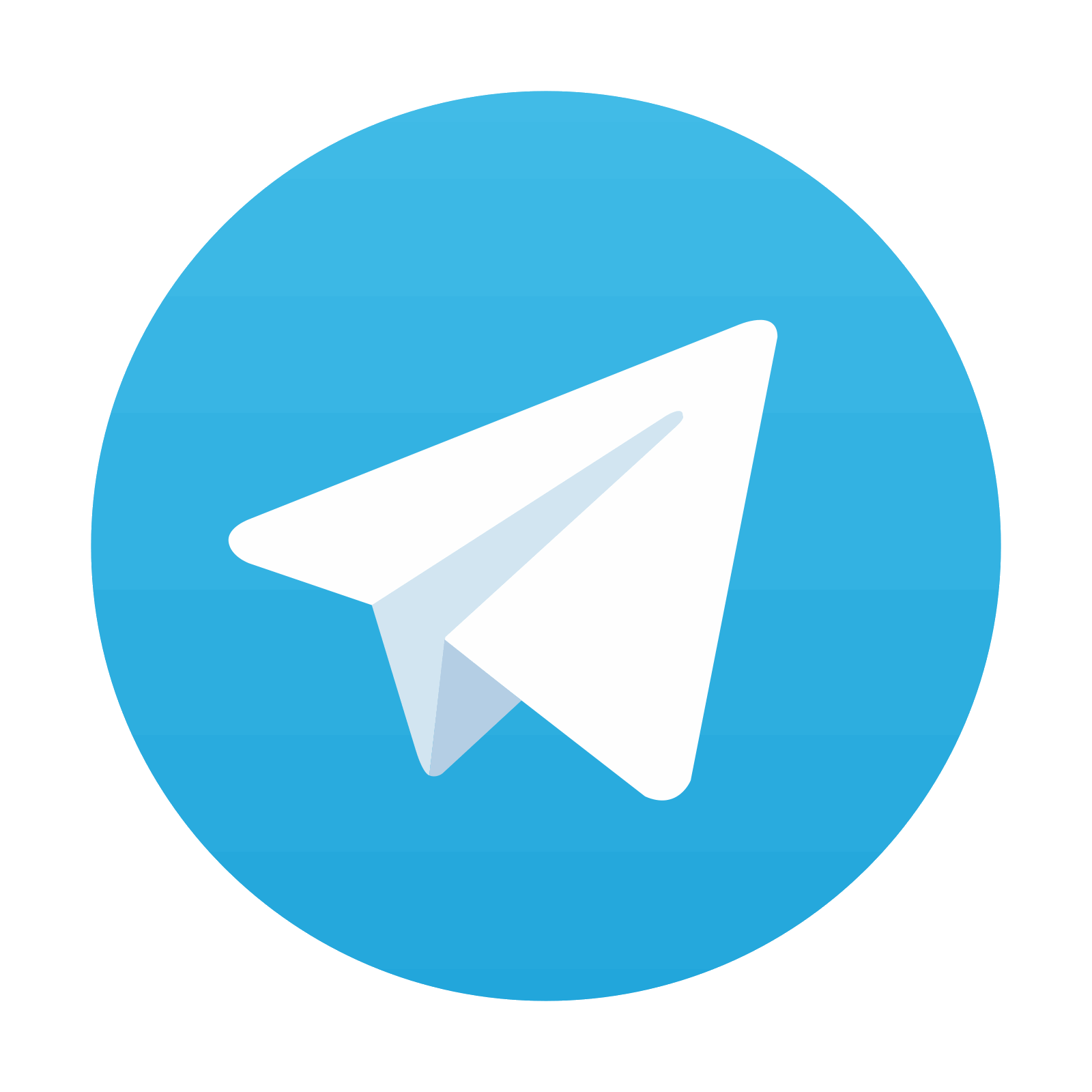
Stay updated, free articles. Join our Telegram channel
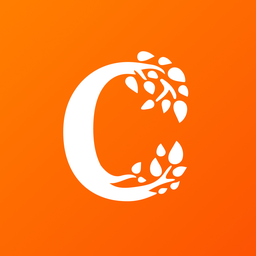
Full access? Get Clinical Tree
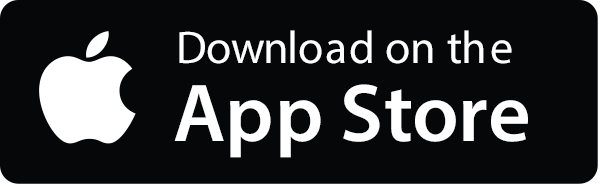
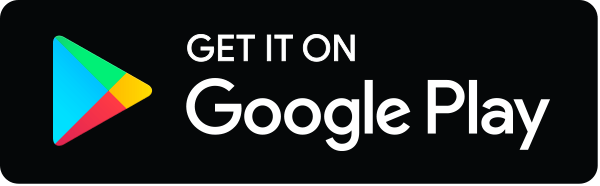