Key Points
- ▪
All theories of facial growth control mechanisms are speculative, and none is fully satisfactory.
- ▪
In Moss’s functional matrix hypothesis, the functional matrix surrounding cartilage and bone regulates the growth of cartilage and bone.
- ▪
The craniofacial complex can be divided into three regions: the neurocranium, nasomaxillary complex, and mandible.
- ▪
Asymmetry is recognized as an intrinsic facial characteristic.
- ▪
Ionizing radiation negatively affects developing bone and soft tissue, causing several side effects and facial alterations, both functional and cosmetic.
- ▪
The correlation between animal and human experiments regarding the effects of nasoseptal and sinus surgery on craniofacial growth remains unclear.
- ▪
Debate is ongoing regarding the correction of nasal septal deformity or deviation in neonates and older children. A conservative approach may be warranted until more definitive data are available.
- ▪
Controversy exists in the literature as to the role of nasal or nasopharyngeal obstruction in dentofacial growth and development.
- ▪
Pierre Robin sequence may occur as an isolated entity or as part of several craniofacial syndromes.
- ▪
Craniofacial surgery in patients with mandibulofacial dysostosis (Treacher Collins syndrome) using osteotomies and advancement techniques appears to assist in the orientation of subsequent growth in a more normal direction and to enlarge the airway at the same time.
- ▪
Choanal atresia is defined as congenital stenosis of the posterior nasal apertures and seems also to present with an overall reduced development of the nasal complex.
- ▪
Effects of functional endoscopic sinus surgery (FESS) on facial growth are yet to be consistently proven. If FESS is indicated, a mini-FESS is recommended to exclusively address the ostiomeatal complex and minimize any potential disturbance in facial growth.
- ▪
The use of resorbable hardware systems in pediatric craniofacial surgery is controversial. Recent evidence does not suggest that resorbable plates are as effective as titanium plates.
Volumes of literature have been written on facial growth and development, and entire academic careers have been spent researching various concepts and theories. Searching through the published literature can be overwhelming. This chapter provides a place to begin the study of facial growth and development and reviews major changes in growth from birth to late adolescence. Growth refers to an increase in size, whereas development indicates an increase in complexity of structure and function.
An understanding of the abnormal first requires comprehension of the normal. Accordingly, this chapter is divided into two parts. A brief review of the main theories of postnatal craniofacial growth and the characteristics of normal postnatal facial growth and development is presented first. Subsequently, abnormal facial growth and development are examined through several clinical examples of relevance in pediatric otolaryngology.
Theories of Craniofacial Growth
Postnatal facial growth appears to be a complex, multifactorial phenomenon. It is clear that genetic factors play a significant role in basic facial pattern, given the resemblance of children to their parents in both facial structure and soft tissue development. However, “environmental” factors most certainly influence facial growth as well. These factors can range from intercellular influences to biomechanical forces (i.e., physical forces acting on bone). As stated in Wolff’s law (1870), “changes in the function of a bone are attended by alterations in its structure.” Bone is a tissue that remodels and responds to the stresses placed on it.
The genetic and environmental control mechanisms of postnatal facial growth and development are poorly understood. The three main theories of postnatal facial growth control include the genetic preprogrammed theory, the cartilage-mediated growth theory, and the functional matrix hypothesis, none of which is fully satisfactory.
Genetic Preprogrammed Theory (Growth Sites)
Early theorists believed that the sizes and shapes of bones were genetically determined within their cells and that sutural patterns were therefore predetermined. These pioneers theorized that because bone size and shape are defined by sutures in the head, the position of sutures limits and controls the expansion of bones. Baume described “growth centres” as places of endochondral ossification with tissue-separating force, resulting in an increase in skeletal mass; “growth sites” were defined as regions of periosteal or sutural bone formation and modeling resorption that are adaptive to environmental influences. The cells of the sutures were thought to grow into the void created when the bone segments pulled apart from the tissue-separating force. In other words, growth sites were proposed to be where growth literally takes place, and growth centers were areas in which genetically predetermined growth takes place. This theory was disproven by experiments involving suture removal and transplantation, which concluded that sutures are not primarily responsible for growth.
Cartilage-Mediated Growth
In 1953, Scott theorized that the cartilage of the nasal septum “is an extension of the cartilage of the cranial base.” As it grows, the cartilage of the nasal septum separates the facial bones from one another and from the cranial portion of the skull. He held that the nasal septum and also the mandibular condyles and synchondroses (otherwise known as cephalic cartilages) are growth centers, allowing growth to take place at the sutures by the ordinary mechanism of surface deposition. As the nasal septum grows, it puts tension on the sutures of the nasomaxillary complex, thereby displacing it forward and downward, with resultant growth occurring at pressure-related growth sites. Tension occurring in the maxillary sutures causes new bone growth when the sutures are pulled apart. Therefore, suture growth is passive and secondary.
Latham theorized that a septomaxillary ligament (connection between the septum and maxilla) in the fetus causes the maxilla to be pulled forward by the septum as the maxilla grows. Animal experimentation involving removal of septal cartilage shortly after birth or secondary to trauma seems to result in midface hypoplasia, but such experiments cannot clearly prove that the cartilage removal causes the growth deficiency. Cartilage seems to play some role that has not yet been precisely determined, but it is otherwise untested and unknown whether a septomaxillary ligament plays a role in postnatal growth.
The Functional Matrix Hypothesis
The most fundamental dictum of the functional matrix hypothesis was stated by Moss as follows:
The origin of all skeletal units, all of their subsequent changes in size, shape and location and their subsequent maintenance and being, are always, and without exception, secondary, compensatory and mechanical obligatory responses to the morphogenetically and temporally prior and primary alterations of the operational (functional) demands of their specifically related functional matrices. Put more succinctly, “Bones do not grow, they are grown.”
The basic premise of this theory is that bone and cartilage do not regulate their own growth. This theory proposes that craniofacial bony growth occurs as a reaction to—or in response to—the functional matrix surrounding it. A functional matrix consists of the cells that comprise muscle, soft tissue, teeth, glands, and nerves, as well as the functioning volumes of various cavities associated with the head and neck region (e.g., nasal, orbital, oral, pharyngeal, and neurocranial). A feedback system exists between the functional matrix and the bone and cartilage involved. Growth of cranial size occurs in response to growth of the brain, growth of the bony orbits occurs in response to the size of the orbital tissues, and growth of the facial bones occurs in response to growth of the surrounding soft tissues. The resultant enlargement of the associated cavities of the head and neck occurs because of the functional demands of airway, breathing, and eating “spaces.” The craniofacial bones are in a dependent relationship with the surrounding soft tissues. Critics maintain that Moss’s theory explains what happens during growth but does not explain how it happens. Moss’s theory is quite useful in clinical practice because it allows separate assessment of each functional unit in the craniofacial skeleton (brain = cranium, eye = orbit, jaws [mandible] = mastication, nasomaxillary complex and associated airways = respiration, and the ear = temporal bone). The functional matrix operates within the genetic constraints of the cells and tissues involved.
Facial growth involves intimate morphogenetic and biomechanical relationships among all of its component tissue parts, each influenced by and in turn influencing the other. A fundamental principle is that no part is developmentally independent or self-contained.
At this time, all theories of growth control mechanisms are speculative, with strengths and weaknesses in each. Further research is required before any definite conclusion can be made.
Bone Growth Concepts
Osteogenesis
The initial step in osteogenesis is the laying down of primary or woven bone. Primary bone is immature bone defined by random orientation of collagen fibers and is temporary, except in a few locations such as the tooth sockets and sutures of the cranial bones. Primary bone generally either becomes secondary (lamellar) bone or is removed to leave bone marrow cavities. Secondary bone differs from primary bone in that its collagen has an orderly pattern, and it has an increased number of osteoblasts. Osteogenesis occurs through intramembranous ossification in mesenchyme and endochondral ossification in preexisting cartilage.
Intramembranous Bone Formation
During intramembranous bone formation, mesenchymal cells differentiate into osteoblasts and accumulate in the area where bone is laid down. The osteoblasts begin to produce osteoid, which consists of collagenous fibers and a homogeneous matrix that is primary bone. Secondary bone replaces the primary bone through ossification of the osteoid.
Endochondral Bone Formation
In endochondral bone formation, simultaneous removal of cartilage and subsequent formation of bone occur. Endochondral bone formation is more complex than intramembranous bone formation. Various zones exist in active endochondral bone formation, including the zones of reserve cartilage, proliferating cartilage, hypertrophic cartilage, calcified matrix, and resorption. The hypertrophic cartilage cells enlarge, and the surrounding matrix becomes calcified. Periosteal (or, more precisely, perichondral) bone forms as a separate collar of bone around the circumference of the center of this cartilage bar. A vascular bud migrates into the cartilage, and a primary marrow cavity forms. Meanwhile, activity at the various zones continues. Erosion of the cartilage takes place at the two ends of the expanding primitive marrow cavity, and bone forms on the spicules of the cartilage at the erosion front. The epiphyseal plate of long bones allows simpler visualization of this process ( Fig. 6-1 ).

Remodeling and Displacement
Enlow and Hans described the two basic types of growth movement, remodeling and displacement, which pertain to both bone and the surrounding soft tissues in the craniofacial complex. Remodeling involves changes in the size and shape of bone, the joining of separate bones and how they fit together, the adaptation intrinsically and extrinsically of these newly joined bones, and finally the relocation of these bones—each influenced by their overall enlargement. Displacement is the process whereby a space is created between bones as they enlarge. Resorption and deposition are ongoing throughout the craniofacial complex during growth as part of the remodeling and displacement processes.
According to Rodan, “bone formation and bone resorption are finely regulated processes, controlled by local and systemic factors, carried out by a family of different interacting cells which communicate via cytokines, cell contacts, and elaboration of matrix which feeds back on cellular behavior.”
Facial Changes during Normal Growth and Development
General
The faces of infants and children clearly differ from those of adults. Matching an infant’s photograph with that of the corresponding adult photograph is challenging because of the changes that occur with growth and development of the craniofacial region. A baby’s face is pudgy and round, relatively wide, and vertically short; the nose is small; the eyes appear relatively far apart; and there is a prominent forehead.
Infants have a large head relative to their body size. Their facial complex, which includes the nasomaxillary complex and mandible, is much smaller than the calvaria and basicranium ( Figs. 6-2 through 6-4 ). The size ratio of the cranium to the face changes with growth and development of the facial complex. In infants the cranium-to-face ratio is approximately 3 : 1, whereas in adults it is approximately 2 : 1 (see Fig. 6-4 ).



With growth, increasing demands are made on the respiratory and masticatory systems. As part of the growth of the facial complex, the nose, mandible, and maxilla increase in size—resulting in the larger, coarser features of the adult face in comparison with the child’s (see Fig. 6-3 ). The facial complex increases in size relative to the cranium, as discussed earlier. Similarly, body size increases relative to head size, to result in normal adult body and head proportions ( Fig. 6-5 ).

Craniofacial Regions of Growth
The craniofacial complex can be divided into three regions: (1) the neurocranium, which is further divided into the calvaria and basicranium; (2) the nasomaxillary complex; and (3) the mandible. Figures 6-2 and 6-3 show the changes that occur in the craniofacial complex from birth to adulthood. The embryonic human skull has 110 ossification centers, many of which fuse to result in 45 bones in the neonatal skull and ultimately 22 bones in the young adult skull. Table 6-1 summarizes the changes in size of various anatomic sites that take place during growth and development from infancy to adulthood.
Growth Aspect | Age/Development |
---|---|
Neurocranium growth | Birth/25% of ultimate growth |
6 months/50% of ultimate growth | |
2 years/75% of ultimate growth | |
10 years/95% of ultimate growth | |
Facial skeletal growth | 10 years/65% of ultimate growth |
Neurocranium/face size ratio | Birth/8 : 1 |
2 years/6 : 1 | |
5 years/4 : 1 | |
Adult/2.1 : 1 to 2.5 : 1 | |
Brain size | Birth to adulthood/3.5× increase |
Fontanelle closure | 3 months after birth/anterolateral |
Second year of life/posterolateral | |
2 months after birth/posterior | |
Second year of life/anterior | |
Orbits | 2 years/50% of ultimate growth |
7 years/100% of ultimate growth | |
Inner ear | 6 months of fetal life/full adult size |
Brain weight | Full-term infant/25% of eventual weight |
6 months/50% of eventual weight | |
2 years/80% of eventual weight | |
10 years/almost eventual weight | |
Postnatal brain growth | 1 year/60% of ultimate growth |
2 years/80% of ultimate growth | |
8 years/95% of ultimate growth | |
Minimal growth continues to at least age 25 years | |
Facial height (males) | Birth/40% to 61% of ultimate growth |
Upper facial height | 1 year/63% to 86% of ultimate growth |
3 years/72% to 90% of ultimate growth | |
5 years/82% to 100% of ultimate growth (mean, 89%) | |
Total facial height | Birth 38% to 45% of ultimate growth |
1 year/50% to 66% of ultimate growth | |
3 years/66% to 78% of ultimate growth | |
5 years/68% to 82% of ultimate growth (mean, 77%) | |
Facial dimension | 3 months/equivalent to 40% of adult size |
2 years/equivalent of 70% of adult size | |
5 years/equivalent of 80% of adult size | |
Nasal height and nasal bridge length | Fully mature in boys at age 15, girls at age 12 |
Nasal tip protrusion | Boys age 15, females age 13 |
Upper and lower nasal dorsum | Males age 14, females age 12 |
Anterior and posterior nasal depth | Males age 14, females age 12 |
Foramen magnum | 1 year/80% of transverse adult size |
1 year/74% of sagittal adult size | |
Skull base (basion = nasion) | Birth/56% of adult length |
2 years/70% of adult length | |
10 years/90% of adult length | |
Prechordal part contributes 75% of the postnatal length and parachordal (basioccipital) contributes to 25% | |
Dentition eruption | 8 months to 2.5 years/primary teeth |
6 years to 20 years/permanent teeth | |
Maxillary antrum height | Birth/20% |
7 years/50% | |
10 years/55% | |
Bizygomatic width | Birth/60% |
3 years/80% | |
5 years/82% | |
10 years/85% | |
Cranial width | Birth/65% |
3 years/85% | |
5 years/92% | |
10 years/95% | |
Cranial length | Birth/60% |
3 years/85% | |
5 years/90% | |
10 years/95% |
Neurocranium (Calvaria = Roof and Basicranium = Floor)
Calvaria.
The calvaria comprises the frontal, parietal, and parts of the temporal, occipital, and sphenoid bones. These bones are formed by intramembranous ossification, with the exception of noncalvarial parts of the occipital, temporal, and sphenoid bones, which are formed through endochondral ossification. These bones initially are separated by fontanelles, which mature into sutures early in development and then finally fuse in adult life ( Fig. 6-6 ).

As the child’s brain grows, the calvarial bones are displaced, which theoretically results in the deposition of new bone on the sutural edges. Growth of bone is seen on both the internal and external tables of the calvaria, leading to an expanded medullary space. Significant thickening occurs from infancy to adulthood. The ascendancy (dominance) of the neurocranium over the facial complex during the early stages of life is clearly related to the inordinately early development of the brain. The major portion of calvarial growth is completed by the age of 4 years ( Fig. 6-7 ).

Basicranium.
Growth of the cranial base creates space for the enlarging brain and oronasal structures with their associated airways. The basicranium grows more slowly than the neurocranium. The cranial base exhibits 63% and 95% of its ultimate size at birth and 10 years of age, respectively. The basicranium comprises parts of the occipital, temporal, sphenoid, and ethmoid bones. The occipital, temporal, and sphenoid portions form through endochondral ossification. The ethmoid and inferior nasal concha form solely by intramembranous bone formation.
Synchondroses are present in the midline ( Fig. 6-8 ) and remain after the chondrocranium (cartilage precursor of the basicranium) has ossified. During childhood, the spheno-occipital synchondrosis is the principal growth cartilage of the basicranium, and its growth persists into early adolescence. The other synchondroses of the basicranium (the sphenoethmoidal, intersphenoidal, sphenofrontal, and frontoethmoidal) all play a minimal role in postnatal growth. The synchondroses have zones similar to those of the epiphyseal cartilage of long bones; however, growth occurs in two directions, resulting in elongation of the basicranium until its ultimate ossification is completed ( Fig. 6-9 ).


Computed tomography (CT) data have been used to document the development of ossification center sutures and synchondroses in the chondrocranium throughout childhood and to provide standards for skull base maturity. In addition, both CT and magnetic resonance imaging have been used to characterize postnatal normal variation of the cranial base.
In newborns and infants, the cranial base is quite flat. With growth into childhood, a more convex superior or flexed appearance emerges, with further enlargement during adolescence and adulthood. The spheno-occipital synchondrosis becomes more flexed, as the maxillary portion of the nasomaxillary complex moves toward the foramen magnum and under the anterior cranial fossa ( Fig. 6-10 ). Components of the cranial base can be seen more clearly in skulls of older children than in those of infants. The foramen magnum approaches adult size, and the primary dentition has erupted by 3 years of age. By 8 years of age, the occipital bone has fused around the foramen magnum, and the secondary dentition is erupting. The major difference between the adolescent and adult skull is fusion of the spheno-occipital synchondrosis. Fusion of this synchondrosis begins at approximately 15 years of age for boys and at 12 or 13 years of age for girls on the cerebral surface. Ossification on the external aspect is complete by age 20 ( Fig. 6-11 ). The chondrocranium-basicranium is a junction between both the neurocranial and facial complex bones. Its alteration affects not only neurocranial and skull growth but also facial morphology.


Nasomaxillary Complex
The nasomaxillary complex develops through intramembranous ossification. It includes the nasal, lacrimal, maxillary, zygomatic, pterygoid, and vomer bones.
The nasomaxillary complex is displaced forward and downward from the cranial floor (basicranium) as a result of growth of the associated soft tissues. New bone growth occurs at sutural contact surfaces on both sides of the suture, with a resultant increase in the size of both bones involved ( Fig. 6-12 ). Bone is added at the frontal maxillary, zygomaticotemporal, zygomaticosphenoidal, zygomaticomaxillary, ethmoidomaxillary, frontoethmoidal, nasomaxillary, nasofrontal, frontolacrimal, palatine, and vomerine sutures. Interaction between the basicranium and the nasomaxillary complex constantly occurs. Rotatory displacement is caused by the downward and forward growth of the middle cranial fossa, resulting in greater or lesser amounts of displacement and remodeling growth in the posterior and anterior parts of the maxilla, depending on the biomechanical forces involved.

The anterior surface of the maxilla is an area of significant resorption ( Fig. 6-13 ); nonetheless, the maxilla still grows forward, exemplifying remodeling and displacement ( Fig. 6-14 ). Growth and development of the nasomaxillary complex are shown in Figure 6-15 . Vertical nasomaxillary growth occurs at the frontomaxillary, frontozygomatic, frontonasal, ethmoidomaxillary, and frontoethmoidal sutures as a result of orbital and nasal growth.



The orbits, which are linked to cranial growth, are quite large at birth because of their relationship with the globe. Orbital remodeling is quite complex; 50% of postnatal orbital growth is achieved by the age of 2 years, and it is almost complete by age 7 years. The superior aspect of the orbit in toddlers is similar to that in adults, although the inferior aspect differs. The floor of the nose is almost at the level of the inferior orbital rim in infants; the distance progressively increases, such that the floor is significantly lower in adults. The eyes in the adult face are not much farther apart than those of a child. However, as explained by Enlow and Hans, “because of the larger nose, higher nasal bridge, increase in the vertical facial dimension and the widening of the cheekbones, the eyes of the adult appear much closer together.” The main contribution to the widening face is from the lateral expansion of the zygomaticomaxillary sutures and the intermaxillary suture growth. Eruption of the primary and secondary teeth results in an increase in the size of the maxilla. As the maxilla moves downward and forward and secondary teeth erupt, the maxillary sinuses increase in size as well.
Sinuses
At birth, the maxillary sinus is large enough to be seen radiologically. Relatively rapid growth occurs between ages of 1 and 4 years; by age 5 years, the sinus has started to expand laterally beyond the infraorbital foramen, although its floor does not extend below the nasal cavity. As the permanent dentition erupts, there is growth inferiorly of the floor of the sinus. By the age of 16 years, the maxillary sinus reaches full size ( Fig. 6-16 ). The ethmoid sinuses are present at birth and have attained adult size by the early teens. The frontal sinus can be distinguished from the ethmoids around age 5 years and demonstrates a variable growth rate until reaching adult size in late puberty. The sphenoid sinus is the last to develop and is present radiologically at approximately age 6 years. Growth of the sphenoid sinus continues well past puberty into the early adult years.

Shah and coworkers reviewed 91 CT scans in 66 patients (16 obtained serially over time) between birth and 12 years of age and demonstrated periods of significant growth in the paranasal sinuses in children. The ethmoid sinuses were the first to fully develop, followed by the maxillary, sphenoid and frontal sinuses.
Mandible
The mandible forms in relation to the first branchial cartilage, otherwise known as Meckel’s cartilage. Meckel’s cartilage is interesting in that it is not replaced by bone. Instead, membranous bone forms lateral to Meckel’s cartilage, and Meckel’s cartilage then disappears ( Fig. 6-17 ). At birth, the mandible comprises two bones with the symphysis menti between them. This suture closes by the age of 1 year, after which the major portion of mandibular growth occurs posteriorly.

Resorption occurring anteriorly and deposition occurring posteriorly result in the overall growth of the mandible ( Fig. 6-18 ). Although the condyles are a major site of growth, they are not a master growth center, as was previously thought.

Mediation of endochondral bone growth occurs at the articular contact portion of the condyle. The main contribution of condylar cartilage is to provide regional adaptive growth by maintaining the condylar area in a proper anatomic relationship with the temporal bone as the whole mandible is simultaneously being carried downward and forward. The mandible grows by enlarging and widening in response to the increase in size of the muscles of mastication and an enlarging oropharynx, which accommodates the concomitant growth of the nasomaxillary complex. The ramus grows posteriorly and at the same time increases the inter-ramal dimension along with the laterally expanding cranial floor. The coronoid processes grow superiorly and buccally, with the anterior edges of the rami being continually resorbed in the process. The condyles grow posteriorly, superiorly, and laterally ( Fig. 6-19 ). Even though it is a single bone, the mandible has been described by Sperber as six functional skeletal subunits. These subunits are the alveolar, coronoid, and angular bones, the condylar process, and the chin, all of which are attached to the “body” of the mandible. According to Moss’s concept, the functional matrix of each subunit then influences its growth (teeth: alveolar unit; action of the temporalis muscle: coronoid process; masseter and medial pterygoids: angle and ramus; lateral pterygoid–condylar process, perioral muscles, tongue, and expanding oropharyngeal cavity: entire mandible).

The infant mandibular arch is U-shaped, whereas the adult arch is more V-shaped ( Fig. 6-20 ). The gonial angle (the angle between the ramus and the body) is more obtuse in infants than in adults ( Fig. 6-21 ). Mandibular growth takes place in two specific growth spurts, the first between the ages of 5 and 10 years and the second between ages 10 and 15 years. Growth in mandibular width is completed during early adolescence. Growth in mandibular length is complete 2 to 3 years after menstruation in females and approximately 4 years after attainment of sexual maturity in males. Growth in mandibular height is complete in the late teens in females and early twenties in males (see Fig. 6-21 ).


Internal and external rotational changes of the mandible occur during growth and, of course, in conjunction with rotational changes in the maxilla ( Fig. 6-22 ). Bjork and Skieller described this in detail using metallic implants. Miller and Kerr supported their findings but used a digitizer program for longitudinal follow-up with cephalograms in a number of patients. Overall mandibular growth is not uniform or steady, because corpus length and ramus height grow in an irregular pattern. Jaw rotation influences the degree of tooth eruption.

Craniofacial cephalometric and anthropometric studies are abundant in the literature, and normative data are available. A review of these topics is beyond the scope of this chapter; however, these measurements may be used to assist in the interpretation of optimal growth and development in the clinical situation.
Abnormal Facial Growth and Development
Asymmetry of the human face is well recognized as a normal finding and in fact is an intrinsic facial characteristic. According to Ferrario and colleagues, “the skeletal structures show a greater degree of asymmetry than their soft tissue drape.” Age is certainly one factor that influences asymmetry. Melnik found that the left side of the face was larger at the age of 6 years, whereas the right side of the face was larger at age 16. Disagreement exists in the literature regarding the degree, side, and localization of normal facial asymmetry, which some attribute to methodologic differences. Normal facial asymmetry involves many subtle differences that are not readily apparent to the viewer. Conversely, abnormal facial asymmetry typically is quite obvious.
Physical attractiveness, is to a large extent based on facial symmetry and what is perceived to be “normal.” Langlois found that the preference for attractive persons is evident early in life and involves all age groups and races. As a result, individuals whose appearance is divergent from the “norm,” with or without intellectual impairment, are viewed differently in our society.
Abnormal facial growth and development may be of iatrogenic, traumatic, mechanical, or genetic etiology. Recognized causes include radiotherapy, FESS, internal fixation, nasoseptal trauma and surgery, and nasal obstruction as related to dentofacial growth.
Radiotherapy
Ionizing radiation is widely recognized as detrimental to the growth of developing tissue. In a majority of cases, impaired growth results from the application of full doses to radiosensitive tumors. Rhabdomyosarcoma and undifferentiated sarcomas constitute the most common type of malignant tumor of soft tissue of childhood, and 35% are found in the head and neck region. Retinoblastoma is the most frequent malignant ocular tumor in childhood and is a frequent indication for radiotherapy in the pediatric population. Multimodal therapy (radiotherapy and chemotherapy) has been effective in the treatment of these tumors with a 3-year relapse-free survival rate of between 70% and 100%. The value of radiotherapy has not been questioned, given its success rate. However, as more children have been cured of cancer and the survival rates have increased, concerns about the long-term side effects of these therapeutic regimens (alone or in combination) have arisen.
The effects of radiation therapy on facial growth, as well as radiation’s collateral effects, are detailed in Table 6-2 . Virtually 100% of children who have received radiotherapy suffer from alterations in the development of facial soft tissues, and in more than 60% of these children, the facial bones are affected. The younger the patient at the time of irradiation, the greater the effects on craniofacial development. These side effects are more frequent and more severe within the first 10 years after irradiation.
Category | Manifestations |
---|---|
Skin | Dryness, abnormal pigmentation, telangiectasias, malignant degeneration, atrophy, alopecia, sebaceous cysts |
Mucosa | Xerostomia, atrophy, fibrosis |
Soft tissues | Atrophy, hypoplasia, sensitivity to infections |
Facial bones | Growth retardation, hypoplasia, dysplasia, appositional growth restriction, weak resistance to infections, loss of cortical bone density, atrophy of alveolar process, micrognathia, facial asymmetry, condylar hypoplasia, “bird face,” abnormal occlusion |
Teeth | Failure to erupt, disturbed eruption, damage to tooth buds, anodontia, hypodontia, pseudoanodontia, mineralization, caries, root foreshortening, enamel dysplasia, atypical root morphology |
Orbit | Bone and soft tissue hypoplasia, persistent infections. |
Eyes | Cataracts, xerophthalmia, retinopathy, corneal lesions, loss of vision, keratoconjunctivitis, dryness of globe, enophthalmos, stenosis of lacrimal duct |
Head and neck muscles | Atrophy, trismus, fibrosis |
Risk of second malignancy | Osteogenic sarcoma, soft tissue sarcomas usually within radiation fields, thyroid, breast, acute myeloid leukemia, histiocytoma |
Brain | Cerebral function damage |
Middle ear | Serous otitis media, chronic otorrhea |
Paranasal sinuses | Bone hypoplasia, chronic sinusitis |
Audiology | Sensorineural or conductive hearing loss |
Speech | Velopharyngeal incompetency |
Hormonal | Growth retardation, growth hormone deficiency |
Psychologic | Psychologic disorders |
Cosmetic | Bone or soft tissue hypoplasia, facial asymmetry, severe neck fibrosis, nuchal asymmetry |
Various | Nutritional and vitamin deficiency |
The optimal dose of irradiation that both minimizes side effects on growing tissue and remains effective in eradicating the cancer is difficult to determine. Techniques of radiotherapy administration have changed significantly during the past 20 years, including radiotherapy with megavoltage, hyperfractionation, three-dimensional therapy, intensity-modulation, and conformal radiation with multiple fields. The principal aim is to achieve a balance between cure and late toxicity of the treatment. In all children undergoing radiotherapy of the head and neck, multidisciplinary follow-up must be part of the ongoing management.
Amifostine has been used efficiently in multiple clinical and research protocols to selectively protect normal tissue during radiotherapy; the mechanism is not completely understood, but animal studies have demonstrated that the cytoprotection provided by amifostine may be mediated through a combination of reduced cellular injury with enhanced promotion of cellular bone rebuilding potential. Experiments in New Zealand white rabbits have shown that it may be possible to attenuate the deleterious effects of orthovoltage radiation (voltage in the range of 140 to 400 kilovolts) on craniofacial bone growth by pretreatment with amifostine 20 minutes before irradiation is given. Clinical trials will be required to prove its effectiveness and safety in humans.
Functional Endoscopic Sinus Surgery)
Functional endoscopic sinus surgery (FESS) has become the standard of care in the surgical management of chronic sinusitis and other sinus disorders in the pediatric population (see Chapter 17 ). Its effectiveness has been accepted when clear indications for surgery are established. However, concerns remain regarding potential negative effects on the growth and development of paranasal sinuses and the nasomaxillary complex in the pediatric patient. A knowledge of sinus anatomy during different stages of development is imperative for making decisions regarding FESS in the pediatric population. The absolute and relative indications for FESS in the pediatric population have changed through the 1990s and into the new millennium, related to the evolving definitions of sinus disorders and short- and long-term outcome measures.
Although the relationship between nasal breathing and sinus development remains unclear, it is generally accepted that the development of the paranasal sinuses is linked to the growth of the nasomaxillary complex. Therefore, it is a reasonable assumption that any intervention in a growing patient, whether open or endoscopic, may have negative effects on the normal process of remodeling, expansion, and pneumatization.
Sinus hypoplasia may result from primary causes related to individual development or secondary causes that affect the activation of bone formation factors or the removal of growth centers. Several retrospective studies have examined the effects of facial trauma, chronic sinusitis, or FESS in children, with often conflicting results. The uncinate processes and ethmoids of 84 children (mean age, 6 years; age range, 1 to 18 years) who previously had undergone FESS were studied. In children younger than 9 years of age, woven and lamellar bone were present, whereas children older than 9 years of age had exclusively lamellar-mature bone. These results suggest that the safest age at which a pediatric patient should be operated on is 9 or older. In a study of five children (mean age, 30 months) who had undergone bilateral FESS for refractory chronic sinusitis, four children showed unilateral maxillary hypoplasia and one child showed bilateral hypoplasia without apparent changes in facial symmetry at a mean postoperative time of 42 months. In another study, no long-term significant differences in sinus volumes in eight patients (age range, 2 to 14 years) who had required FESS after unilateral orbital complications of acute sinusitis were found, but an association with age could not be determined due to the wide age range of the patients studied. In a retrospective study, there was no evidence of facial growth disruption found 10 years after FESS in 67 children between 2 and 4 years of age. Although the extent of the surgery varied among patients, most underwent antrostomy enlargement and bilateral anterior ethmoidectomy. Finally, in a small series of 14 patients with cystic fibrosis ranging in age from 9 to 28 years, there was no impact on the outcome of facial growth in comparison with a control group of 5 patients with cystic fibrosis who did not require surgery.
Results of animal studies have varied depending on the species and the intervention. In one study, in which 36-day-old New Zealand white rabbits had a unilateral maxillary sinus filled with polyhydroxyethylmethacrylate, there was no adverse effect on craniofacial growth. Likewise, controlled resection of the bony sinonasal wall and partial resection of the anterior ethmoid did not affect later development of nasal bones and maxilla on 20 young New Zealand white rabbits. Conversely, significant reduction of the facial growth and maxillary-ethmoidal sinus development was evident at 6 months after surgery on the operated side, following unilateral FESS in eight newly weaned piglets. Similarly, significant restrictive effects on piglet facial skeletal development were found to result after FESS.
Keeping in mind the small sample sizes in several studies and the lack of prospective studies, concerns still remain regarding FESS in the pediatric population. Prudence is recommended regarding the timing and extent of FESS in children, and clear indications for surgery should be present. Unless the patient has an acute complication of sinus origin, FESS for chronic sinusitis should ideally be delayed until maximal medical therapy has been undertaken in order to avoid potential negative effects on facial growth. When surgery is necessary, a conservative procedure such as the mini-FESS is strongly recommended in order to preserve as much as possible of the normal mucosa, periosteum, and bone. The mini-FESS procedure involves removing the inferior uncinate process and opening the maxillary ostium and anterior ethmoid bulla. Thus this procedure addresses the primary area of outflow obstruction, the ostiomeatal complex (uncinate process and infundibular area surrounding the anterior ethmoid cells). Further prospective studies in both animal and humans are required to clarify the role of FESS in the facial growth of children.
The use of balloon catheter sinuplasty (BCS) technology is a recent advancement that aims to preserve mucosa and the anatomy surrounding the natural ostium of the paranasal sinuses. A balloon-tipped catheter is placed into the opening of the sinus, followed by balloon inflation to radially widen the natural ostia. Theoretically, BCS is a minimally invasive and less traumatic procedure that directly addresses the natural opening of the various sinuses. A recent retrospective cohort comparing the efficacy of BCS with ethmoidectomy in comparison to traditional FESS in children with chronic rhinosinusitis showed both procedures to have comparable efficacy in the resolution of sinus symptoms. Although both interventions appear safe and feasible in the pediatric population, it will be interesting to evaluate whether there is a long-term additional advantage to BCS by evaluating differences in the craniofacial growth of children undergoing this procedure in comparison to FESS or mini-FESS.
Internal Fixation and Facial Growth
Pediatric fractures are relatively uncommon, likely related to anatomic factors including a decreased volume ratio of facial surface to cranium, the comparatively flexible and soft nature of the skeletal structure, lesser pneumatization of pediatric facial bones, and the support provided by unerupted teeth in the mandible and maxilla. The amount of force needed to overcome the elasticity of developing bone is greater, therefore increasing the incidence of more severe comorbid injuries occurring with pediatric facial fractures.
Midface skeletal maturity is complete by approximately age 12 years. Any trauma or reconstructive surgery before this age may adversely affect facial growth. In a descriptive retrospective review of children with surgically treated craniofacial fractures, a majority of cases had a favorable outcome with regard to facial growth and development on long-term follow-up; the exceptions were isolated condylar fractures and patients with complex centrofacial trauma. However, there were significant limitations to this study.
The evidence supporting the use of resorbable plate fixation has been mixed. The benefits over time of having a resorbable implant need to be balanced against the potential inferior stability in comparison to titanium, a factor that becomes particularly important in mandibular fractures that support load-bearing forces. A retrospective study concluded that resorbable plate fixation systems are safe, with results comparable to those of metal plates, and the resorption after healing of the fracture avoided negative effects on the growth and development of the facial skeleton. However, a recent Cochrane review compared the effectiveness of bioresorbable fixation systems with titanium systems for the management of facial fractures; three ongoing trials were identified, two of which were stopped before completion mainly due to complications in the resorbable group. In summary, the findings of these aborted trials do not support equal efficacy for resorbable plates as compared to titanium plates.
The direct effect of plates and screws on facial skeletal development has also been studied in animal models. Kittens that underwent osteotomies in conjunction with different fixation systems displayed significant growth retardation in a craniofacial segment, whereas a comparable study in rabbits found no significant effects. These studies concluded that plates and screws should not cross sutures, because of the risk of synostosis. Osteotomy and fixation in the infant rhesus monkey was found to affect craniofacial growth, and growth deformity in selected craniometric parameters was greater with increased complexity of fixation (e.g., longer plates and more screws).
In piglets, the use of rigid internal fixation in the growing skull resulted in a contour deformity of the calvaria. The microplate and screw became completely incorporated into the bone, suggesting that such fixation should not be used in the infant craniofacial skeleton. A study of canine puppies concluded that absorbable materials did not disrupt bone growth, but osteotomies were not performed in this study. A rabbit model was developed using conventional vitallium hardware after osteotomies without the restriction of routine rigid fixation. This system permitted more normal growth of the rabbit head compared with the rigid fixation system. Experimental osteotomies in rabbits were found to cause severe bony restriction, regardless of the use of rigid plate fixation, supporting the theory that trauma itself affects growth and development. Overall, the validity of studies using animal models has been questioned because of the uncertainty of applicability to humans.
Basic principles should be followed in planning the management of facial trauma in children. Complete clinical and image-guided assessment, early repair of fracture and soft tissue injury, minimal but sufficient surgical exposure, and correct technical application of fixation devices are required to achieve good bone stability without disrupting long-term facial growth. Although conservative management is best in most cases (i.e., nondisplaced frontal, zygomaticomaxillary complex, and mandibular fractures), rigid fixation may be needed in more severe injuries, and care must be taken to avoid compromising future dentition and skeletal growth. It is hoped that acquisition of additional data regarding internal fixation and facial growth in the pediatric population will lead to clarity regarding long-term outcomes.
Nasoseptal Trauma and Surgery
It has long been suggested that nasal trauma or surgery has a detrimental effect on the nasofacial development of growing children. It is important to recognize that nasoseptal deformities are a result of a complex interaction of factors, including trauma, surgery, inheritance, and individual growth patterns. Up to 65% of adults with a deviated nasoseptal complex are reported to have sustained trauma in childhood. Surgical correction of nasoseptal deformities during childhood remains very controversial because of potential adverse functional or aesthetic long-term sequelae.
The differences in thickness of the nasal septum in humans may contribute to the distribution and the tendency toward specific fracture lines through the nasal septum. Fracture lines tend to follow the thinnest and most vulnerable parts of the septum. For example, C-shaped septal fractures were first described in adults by Harrison in 1979. The distribution of the fracture lines forming a “C” is characterized by 1) a vertical portion situated in the thin central area of the perpendicular plate, 2) the inferior extension crossing the septo-ethmoidal junction and followed into the thinner zone of septal cartilage, and 3) the superior extension passing the anterior edge of the perpendicular plate and running into the thinner zone just under the nasal dorsum.
Animal Studies
Historically, various animal models of nasal septal trauma have been developed, with many early studies performing destructive procedures that did not replicate surgical approaches used in human patients. Removal of septal cartilage from several different types of newly weaned animals resulted in significantly shortened hard palates on autopsy specimens, suggesting that palatal growth depended on the growth of the nasal septum. The degree of snout deformity in rabbits was proportional to the amount of septum and perichondrium resected. Others asserted that the septum plays a passive role in the development of the midface, acting only as a support structure in guinea pigs and puppies. A later report noted that the septum is not the primary structure determining facial growth in guinea pigs.
Beginning in the 1970s, experimental models changed to more closely approximate surgical procedures performed in human patients. Conservative septoplasties in dogs did not result in growth retardation when the mucoperichondrium was preserved and the removed cartilage was reinserted but not sewn in. Severe deformities in rats occurred after septoplasty, regardless of whether or not there was preservation of the mucoperichondrium. In a study of rabbits, the basal portion of cartilage, the perpendicular lamina, and the junction between cartilage and the premaxilla and cartilage with the sphenoid were identified as the key areas in nasal development. Septal surgery preserving mucoperichondrium in ferrets did not affect facial development. A septoplasty animal model in prepubertal New Zealand rabbits demonstrated underprojection of the snout in comparison with the control group, despite preservation of wide, strong and intact dorsal and caudal septal struts with continuously attached mucoperichondrium on one surface. Interestingly, this animal model included a group of rabbits that underwent stenting of the septal defect with a porous polyethylene graft, but prevention of snout underdevelopment could not be demonstrated. In summary, it remains difficult to correlate findings in animal studies with outcomes in humans.
Clinical Studies
In human studies it is difficult to determine the contribution of surgical treatment of trauma versus the trauma itself to the observed adverse effects on nasofacial growth and development. A number of authors have theorized on the growth centers of the septum( Fig. 6-23 ). However, the long-term effects on nasal growth may be difficult to predict. Septal abscess is a known risk factor for permanent nasal deformity such as a saddle nose. However, even patients with a septal abscess at a young age ( Fig. 6-24, A ) may at maturity have a normally proportioned nose ( Fig. 6-24, D ).
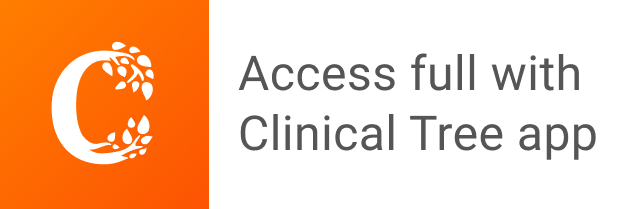