Overview
Phototransduction is the series of biochemical events that leads from photon capture by a photoreceptor cell to its hyperpolarization and slowing of neurotransmitter release at the synapse. This overall process includes an activation phase and a recovery phase. While the fundamental mechanisms of phototransduction are constant over a wide range of light intensities and very similar in rods and cones, the quantitative features differ strikingly depending on the level of ambient light (see Chapters 19, 20 ) and the cell type. Although we know more about the biochemistry of phototransduction than we do about any other neuronal signaling pathway, numerous mysteries remain.
Location and compartmentalization of rods and cones
Rod and cone photoreceptors are densely packed in the outermost layer of the neural retina, stretching from their synapses in the outer plexiform layer to their most distal tips, which are embedded in the membrane processes of the retinal pigmented epithelium (RPE) ( Fig. 18.1 ). Photoreceptors are long, thin cells with their long axes aligned along the radii of the eye to maximize light collection. Efficient phototransduction depends upon the organization of photoreceptors into distinct membrane compartments ( Figs. 18.1–18.3 ). Absorption of light and the biochemical cascade of phototransduction are restricted to the outer segment, which is an elongated membrane compartment that is separated from the rest of the cell by a thin connecting cilium or ciliary transition zone. The cilium consists of a bundle of nine microtubule doublets surrounded by a very thin layer of cytoplasm and a plasma membrane. Immediately adjacent is the inner segment, the site of active biosynthesis and oxidative metabolism. Although small molecules and proteins can diffuse along the connecting cilium between the inner and outer segments, this process generally occurs on a slower time scale than that of a light response. This intercompartment transport plays an important role in producing the molecules necessary for phototransduction and modulating their levels, but it does not contribute directly to the phototransduction cascade. Although cytoplasmic diffusion is limited by the constriction at the connecting cilium, this constriction does not pose a major barrier to the propagation of electrical changes along the plasma membrane. Therefore, changes in membrane potential can be passively communicated from the outer segment to the synaptic terminal at the other end of the cell, where they control neurotransmitter release and transmit the signal to higher-order neurons.




The phototransduction cascade takes place in the outer segments. There are two membrane systems in rods ( Figs. 18.3 and 18.4 ). The disc membranes, which can be thought of as flattened sealed vesicles, are stacked up along the long axis of the outer segment and make up the vast majority of membrane surface in the cells. The plasma membrane surrounds the discs and the cytoplasm ( Figs. 18.1e, 18.3, 18.4 ) and forms the interface between the cell and the extracellular space. Although there are proteins linking these two membranes in the outer segment, there does not appear to be flow of lipid or transmembrane proteins between them. In contrast, cones have a single membrane that forms multiple invaginations that resemble discs but are not sealed and are continuous with the plasma membrane. A cone-like relationship between plasma membrane and nascent discs appears to be present at the base of rod outer segments.

The components of phototransduction, discussed in detail further in this section, are primarily localized in the outer segments, and are mostly peripheral or integral membrane proteins. The disc and plasma membranes have distinct protein and lipid compositions.
Dark-adapted rods
The most information at the molecular level is available for the dim light responses of dark-adapted rods (see Chapters 19, 20 ). The cells in this state can respond with amazingly high efficiency to single photon capture events. It is useful conceptually to begin with the resting dark state and then consider the chain of events set in motion when a photon is absorbed.
The resting dark-adapted state
The membrane potential
The following treatment is meant to outline the electrophysiology of rods and cones in biochemical terms; for more details, see Chapters 19 and 20 . In the dark, rods have a resting membrane potential of about –40 mV. The negative value means that there is more positive charge on the outside of the plasma membrane than on the inside. In most resting neurons, the potential is closer to –70 mV, so rods are considered to be relatively depolarized compared with other cells or, as we shall see, compared with fully light-activated rods. In rods, as in other neurons, the source of membrane potential is the inequality of ion concentrations on either side of the plasma membrane generated by the Na + /K + -ATPase. This protein uses the energy released upon ATP hydrolysis to pump sodium ions out of the cell and potassium ions into the cell, and in rods it is found in the plasma membrane of the inner segment (see Chapter 19 ). In most neurons, potassium channels are the predominant conductance at resting potentials, and these allow potassium ions to flow out of the cell down their concentration gradient. Because these channels pass a specific cation, but no anions, a net positive charge accumulates on the outside of the membrane and a net negative charge accumulates on the inside of the membrane. This charge accumulation occurs until the electrical energy stored in the charge separation equals the energy stored in the potassium gradient, the relationship described by the Nernst equation, described in detail in many textbooks. Strictly speaking, this equation only works when the membrane in question contains only a single type of channel permeable to only a single type of ion. In reality, there are always additional minor sources of conductance (other channels and transporters), so that the resting potential is modified by these to give the typical resting value of about –70 mV to –90 mV for excitable cells.
The dark current and the cyclic GMP–gated channel
Rods also have a negative resting membrane potential, but its magnitude is less than in many other neurons because the effects of potassium channels in the inner segment are balanced by a major additional channel current in the outer segment. The lower negative resting potential, or partially depolarized state, of rods is due to the cyclic GMP (cGMP)–gated cation channel (see Chapter 19 ), also known as the cyclic nucleotide–gated (CNG channel), or light-sensitive channel or phototransduction channel (see Chapter 19 ). This multisubunit channel only passes cations, but does not discriminate strictly among different cations, allowing Na + , K + , and Ca 2+ to pass. Among physiologically important cations, it has the highest permeability to Ca 2+ ; however, the much higher concentration of Na + outside the cell (>140 mM, compared with ~1.5 mM for Ca 2+ ) makes Na + the primary carrier of the current through the CNG channel in the dark. Because it tends to dissipate the gradient of charge, by allowing positive charge to enter the cell, the open CNG channel causes the cells to be partially depolarized. Put another way, because there are substantial inward sodium currents in the outer segment to balance outward potassium currents in the inner segment, the resting potential is moved away from the potassium “equilibrium” or Nernst potential of approximately –90 mV, and toward the sodium “equilibrium” or Nernst potential of approximately +60 mV. Because there is net flux of cations out of the inner segment plasma membrane and a net flux of cations into the outer segment plasma membrane, as well as electrical conductance between the inner and outer segments, a complete circuit is made that is known as the circulating current or dark current.
Ca 2+ and the exchanger
The CNG channel also has an influence on the concentration of Ca 2+ , which plays an important role in signaling and regulation in rods and cones, as in other cells. An Na + /Ca 2+ , K + exchanger protein, NCKX2, uses the energy stored in the Na + gradient across the plasma membrane to push Ca 2+ ions outside the cell against their concentration gradient (see Chapter 19 ). In the absence of any inward flux of Ca 2+ , this mechanism would lower the Ca 2+ concentration in the outer segment cytoplasm to about 10 nM. In the dark, there is an inward leak of Ca 2+ through the CNG channel, so the resting level of Ca 2+ is a few hundred nanomolar.
Even though in the dark more channels are open than at any other time, because cGMP levels are at their highest, the percentage of open channels is small; most of the channels are closed even in the dark (see Chapter 19 ). One reason for this situation is that binding of more than one cGMP is necessary to give each channel a high probability of opening, (i.e., the response of the channel to cGMP is nonlinear and shows positive cooperativity). The other reason is that the dark concentration of cGMP is well below the concentration of cGMP at which channel opening probability is 50%, a number that reflects the affinity of cGMP for the channel subunits, as well as the positive cooperativity. Because the dark cGMP concentration is at the low end of the dose-response curve for the channel (therefore, very far from saturation), even a small change in cytoplasmic concentration of cGMP can be immediately sensed as a change in the number of open channels and therefore of the dark current ( Fig. 18.5 ).

Control of [cGMP] by guanylate cyclase and PDE6
The resting, or dark, level of cGMP is determined by the balance between the activity of the enzyme, guanylate cyclase (GC) that synthesizes cGMP and the cGMP phosphodiesterase, PDE6, that degrades cGMP. Both of these have much lower activity in the dark than they do in the light. When fully activated, PDE6 is one of the most efficient enzymes known, and the most active of any of the PDE superfamily of cyclic nucleotide phosphodiesterases. The maximal turnover at saturating cGMP concentrations, k cat , has been reported to be between 1000 s −1 and 7000 s −1 , and the Michaelis constant, the cGMP concentration at which activity is half-maximal, is about 40 μM. Thus, the enzymatic efficiency, k cat /K m , is in the range of 2.5 × 10 7 M −1 s −1 to 1.75 × 10 8 M −1 s −1 , implying that PDE6 operates near the diffusion limit. PDE6 requires two kinds of metal ions for activity. Mg 2+ binds reversibly along with cGMP, and Zn 2+ is permanently bound to high affinity sites essential for catalysis. The other key players in photoactivation are also in quiescent states under dark conditions. The mechanisms for regulation of these enzymes by light are discussed further in this section. GC uses GTP as a substrate and produces cGMP and inorganic pyrophosphate as products. Inorganic pyrophosphate is rapidly broken down by the enzyme inorganic pyrophosphatase, which is found at higher levels in rod outer segments than in any other cell type in which it has been measured. GC is a transmembrane protein that is thought to exist in photoreceptor membranes as a dimer, with each dimer bound to the calcium-binding protein, GC-activating protein (GCAP). PDE6 is a heterotetrameric peripheral membrane protein consisting of two large catalytic subunits, PDE6α and PDE6β, as well as two smaller identical inhibitory PDE6γ subunits. High resolution structures have been determined by cryo-electron microscopy. The catalytic subunits are subject to the set of post-translational modifications associated with isoprenylation, as described further, so the enzyme is bound to the surface of the disc membranes. The PDE6α and PDE6β subunits found in rods are similar in structure to one another, whereas in cones, two identical PDE6α‘ subunits are found. PDE6 catalytic subunits are related in sequence to a large family of cyclic nucleotide phosphodiesterases, the PDE superfamily. These share sequence similarity in their catalytic domains, where the PDE6 isoforms most closely resemble in structure the PDE5 isoforms, which are the targets of sildenafil citrate (Viagra) and other drugs used to treat erectile dysfunction. In addition to the catalytic domains, PDE6 catalytic subunits each contain two GAF domains, one of which contains a noncatalytic binding site for cGMP. Because the total concentration of cGMP-binding GAF domains in the outer segments is about 50 μM, most of the cGMP in the cell is bound to PDE6. The physiologic function of these noncatalytic sites is unclear, but their occupancy is coupled to binding of the catalytic subunits by the inhibitory PDE6γ subunit. It is the PDE6γ subunit that interacts most extensively with the activated form of the G-protein, as described further.
Rhodopsin
In the dark, the photon receptor rhodopsin is in its inactive state with an inverse agonist, 11- cis retinal, covalently attached to its active site ( Figs. 18.6–18.8 ). Ligands that activate receptors are called agonists, those that block the action of agonists are called antagonists, and those that actually reduce receptor activity below the level it has in the absence of any ligand are called inverse agonists. Rhodopsin has seven membrane-spanning helices and is a part of the G-protein–coupled receptor (GPCR) superfamily of signal transducing receptors. Like other GPCRs, its activity consists of its ability to catalyze the activation of a heterotrimeric G-protein. This is the activity of rhodopsin that is inhibited by the chromophore and inverse agonist 11- cis retinal. The apo-form of rhodopsin without a ligand, known as opsin, has much higher G-protein–activating activity than rhodopsin does, a fact that becomes important at high light levels but is relatively unimportant in the dark-adapted state. Rhodopsin is present at very high concentrations in the disc membrane (and somewhat lower concentrations in the plasma membrane), so that about one-third the surface area of the discs is occupied by rhodopsin, and the other two-thirds is occupied by phospholipids, cholesterol, and other minor lipids. Rhodopsin is subject to post-translational modifications that are important for its function. N-linked carbohydrates are found on the domain of rhodopsin that faces the inside of the discs and the extracellular solution, and they appear to be important for proper transport of rhodopsin from its site of synthesis in the inner segment to the outer segment membranes. Two palmitoyl groups are attached via thio-ester linkages to two adjacent cysteine residues near its carboxyl terminus, which tether the polypeptide chain to the cytoplasmic surface of the disc membrane forming a fourth cytoplasmic loop. In addition, the aldehyde moiety of 11- cis retinal and all- trans retinal is not bound to rhodopsin as a free aldehyde, but rather a covalent Schiff’s base linkage is formed between 11- cis retinal and lysine residue 296 in the transmembrane domain of rhodopsin ( Fig. 18.8 ).



The rhodopsin sequence contains 348 amino acid residues. Whereas mutations at only four different positions are found in patients with the disease congenital stationary night blindness (CSNB, see Box 18.1 ), there are over 100 amino acid positions that are found mutated in patients with the blinding disease autosomal dominant retinitis pigmentosa (ADRP, see Box 18.4 ). Therefore, the molecule is not only so sensitive to light that it can become activated by one photon of light, it is also remarkably sensitive to its amino acid composition.
Congenital stationary night blindness (CSNB) comprises a group of genetically and clinically heterogeneous nonprogressive retinal disorders that are mainly caused by defects in rod photoreceptor signal transduction and transmission. Mutations in genes coding for proteins of the phototransduction cascade (RHO, GNAT1, PDE6B, RHOK, SAG, and RDH5) or genes associated with the transmission of the signals from the photoreceptors to bipolar cells (NYX, GRM6, CANCA1F, and CABP4) can lead to this disease.
Night blindness and reduced or absent dark adaptation are typical and early signs of various forms of retinal dystrophies. Forms differ in inheritance pattern (autosomal dominant, autosomal recessive, or X-linked), electroretinograms (ERG; presence or absence of a-wave), refractive error (presence or absence of myopia), and fundus appearance. All patients with CSNB have severely reduced rod ERG amplitudes and many have modestly reduced cone ERG amplitudes ( Fig. 18.9 ). Rod sensitivity in patients is decreased by 100 Å~ to 1000 Å~ compared with normals. Almost all have cone responses with a normal peak implicit time (time interval between the light flash and subsequent peak of b-wave).
Stargardt disease is an autosomal recessive form of juvenile macular degeneration with variable progression and severity. It is caused by mutations in the ABCR (ABCA4) gene on chromosome 1 which encodes a retina-specific ATP-binding cassette transporter protein, in the rims of rod and cone outer segment discs, proposed to serve as a flippase protein for phospholipids conjugated to all- trans retinal. Mutations in this gene have also been attributed to some cases of cone-rod dystrophy, retinitis pigmentosa, and age-related macular degeneration. Pathologic features of Stargardt include accumulation of fluorescent lipofuscin pigments in the retinal pigmented epithelium (RPE) cells and retinal degeneration.
An important fluorophore in lipofuscin is the bis-retinoid pyridinium salt N-retinylidene-N-retinylethanolamine (A2E) formed by the condensation of all- trans retinaldehyde with phosphatidylethanolamine. Significant accumulation of A2E is seen in the RPE of patients with Stargardt disease (Eagle et al., Birnbach et al., ). A2E has several potential cytotoxic effects on RPE cells, including destabilization of membranes, release of apoptotic proteins from mitochondria, sensitization of cells to blue light damage, and impaired degradation of phospholipids from phagocytosed outer segments.
G-protein, G t
Like rhodopsin, the rod phototransduction G-protein, known as transducin or G t ( Fig. 18.10 ), is also found in an inactive state in the dark. For a G-protein, this means that it has GDP, rather than GTP, bound to its α subunit, G tα , and exists in a heterotrimeric form, G tαβγ . The G-protein associates with the disc membranes by virtue of its covalently attached lipids. , A heterogeneous mixture of saturated and unsaturated 12- and 14-carbon fatty acids is found attached to the amino terminal glycine residue of G tαβγ, linked through an amide bond. This reaction occurs during protein translation and is important for G-protein localization in the outer segment. There is a 15-carbon isoprenyl group, farnesyl, attached to the carboxyl terminus of the γ subunit , through a thio-ether linkage. This sort of isoprenylation is found in other phototransduction proteins; in each case it occurs at a cysteine residue four residues from the carboxyl terminus of the initial translation product. The final three residues are cleaved by the action of a protease, and the hydrophobicity conferred by the isoprenyl group is enhanced by the methyl esterification of the carboxyl group on what has been converted into a C-terminal cysteine residue. A prenyl-binding protein, sometimes referred to as PDEδ, binds to the isoprenyl group and acts as a chaperone to help deliver the enzyme in stable form to the outer segment. ,

Importance of lipid milieu
The lipids of the disc membrane play an important role in assembling the phototransduction machinery and providing the proteins a stage on which to act. Rhodopsin and GC are transmembrane proteins, and G t and PDE6 ( Figs. 18.10 and 18.11 ) are peripheral membrane proteins, attached to the membrane by covalently attached lipids. GCAPs are also covalently lipidated by fatty acids on their amino termini, and another phototransduction protein, rhodopsin kinase, is isoprenylated (discussed further in section). The transmembrane CNG channel is found only in the plasma membrane, not in the discs. Experiments with purified proteins have shown that the proper assembly of these proteins on the disc membrane is essential for efficient interactions among them. Rhodopsin diffuses rather rapidly within the lipids of the disc membrane, so that many encounters between it and the G-protein occur every second, even in the dark. Rod outer segment (ROS) membranes have an unusual lipid composition, with a high content of polyunsaturated fatty acids. Close to 40% of the fatty acyl groups of ROS phospholipids are the ω-3 fatty acid, docosahexaenoic acid (DHA or 22:6), which has 22 carbons and 6 double bonds, and DHA can only be synthesized in the body if essential ω-3 fatty acids are consumed in the diet. Cholesterol and cholesteryl esters make up only 10% to 15% of total outer segment lipids but play an important role in establishing the lipid environment in which phototransduction reactions occur.

The activation phase of a light response
Photoisomerization of rhodopsin
Phototransduction begins with absorption of light by rhodopsin ( Figs. 18.6–18.8 ). Rhodopsin has a broad absorption spectrum with a wavelength of peak absorbance close to 500 nm in the green portion of the visible spectrum (discussed further in section). The probability of capture by rhodopsin of a photon of green light passing through the retina is fairly high (~50% for 20 μm long human rods and ~89% for 60 μm long toad rods) due to the large number of discs (~1000) through which the photon must pass and the high concentration of rhodopsin in each rod. When rhodopsin absorbs light, the probability that it will undergo a structural transition, or photoisomerization, is extraordinarily high: 65%. This is one of the most efficient photochemical reactions known, and this efficiency helps explain the exquisite sensitivity of rod cells to a single photon of light. The bound 11- cis retinal chromophore is responsible for the light absorption (polypeptides do not absorb visible light unless they are bound to some chromophoric ligand). In its excited state induced by the light absorption, 11- cis retinal undergoes a photoisomerization at the bond between carbon 11 and carbon 12 in its structure from 11- cis to all- trans retinal ( Fig. 18.8 ). The rearrangement of bonds causes a dramatic change in the structure of retinal, with the new all- trans form representing a straightening out of a kinked molecule. This molecule no longer acts as an inverse agonist locking the opsin protein in an inactive state, but now acts instead as an agonist, or activator of opsin, stimulating it to activate G-proteins.
The retinal chromophore, covalently attached via a Schiff’s base linkage to a lysine residue at amino acid position 296 on rhodopsin (see previously), induces structural changes in the protein upon photoisomerization. After photoisomerization of the chromophore, the protein undergoes a series of conformational changes in the arrangement of its helices to accommodate this event. In other words, when retinal stretches out into the all- trans shape, it pushes part of the protein out of the way. These conformational changes ultimately lead to formation of metarhodopsin II, a significantly different structure than dark rhodopsin ( Fig. 18.7 ). The intermediate conformations of photolyzed rhodopsin can be monitored by high speed and/or low temperature spectroscopy, including UV-visible absorbance, infrared, Raman, and fluorescence spectroscopies. These and other studies have shown that upon light activation, rhodopsin first changes to bathorhodopsin, lumirhodopsin, metarhodopsin I (MI) and metarhodopsin II (MII or R*, the form that activates transducin), and ultimately metarhodopsin III (MIII) before the all- trans chromophore becomes hydrolyzed, leaving the apoprotein opsin. At physiologic temperatures, almost immediately upon photoactivation MI and MII establish a dynamic equilibrium, which can be monitored by the differences in their absorbance spectra. It is MII rather than MI that preferentially binds to and activates the G-protein.
G-protein activation
The key functional difference between rhodopsin and metarhodopsin II is in their interactions with the G-protein transducin (G t ). MII binds to the G-protein much more tightly than does rhodopsin and serves as an efficient catalyst for the activation of G t . G-protein activation occurs about 10 million times faster at high MII concentrations than it does in the dark. The G-protein binds to MII when it is bound to GDP, but rapidly releases the bound GDP ( Fig. 18.9 ). This GDP release is the slow step (~once per 10,000 s) for G-protein activation in the dark but occurs very rapidly when G t is bound to MII. Once GDP is released, the millimolar concentrations of GTP in the cell ensure rapid binding of GTP. Once GTP is bound to the G tα subunit, the G-protein is in its active state. The activated G tα -GTP complex has much lower affinity for both the G βγ subunits and for MII than do either the nucleotide-free state or the GDP-bound state of G tα . One MII molecule can activate many G-proteins in a catalytic fashion at a rate exceeding 150 per second in mammalian rods.

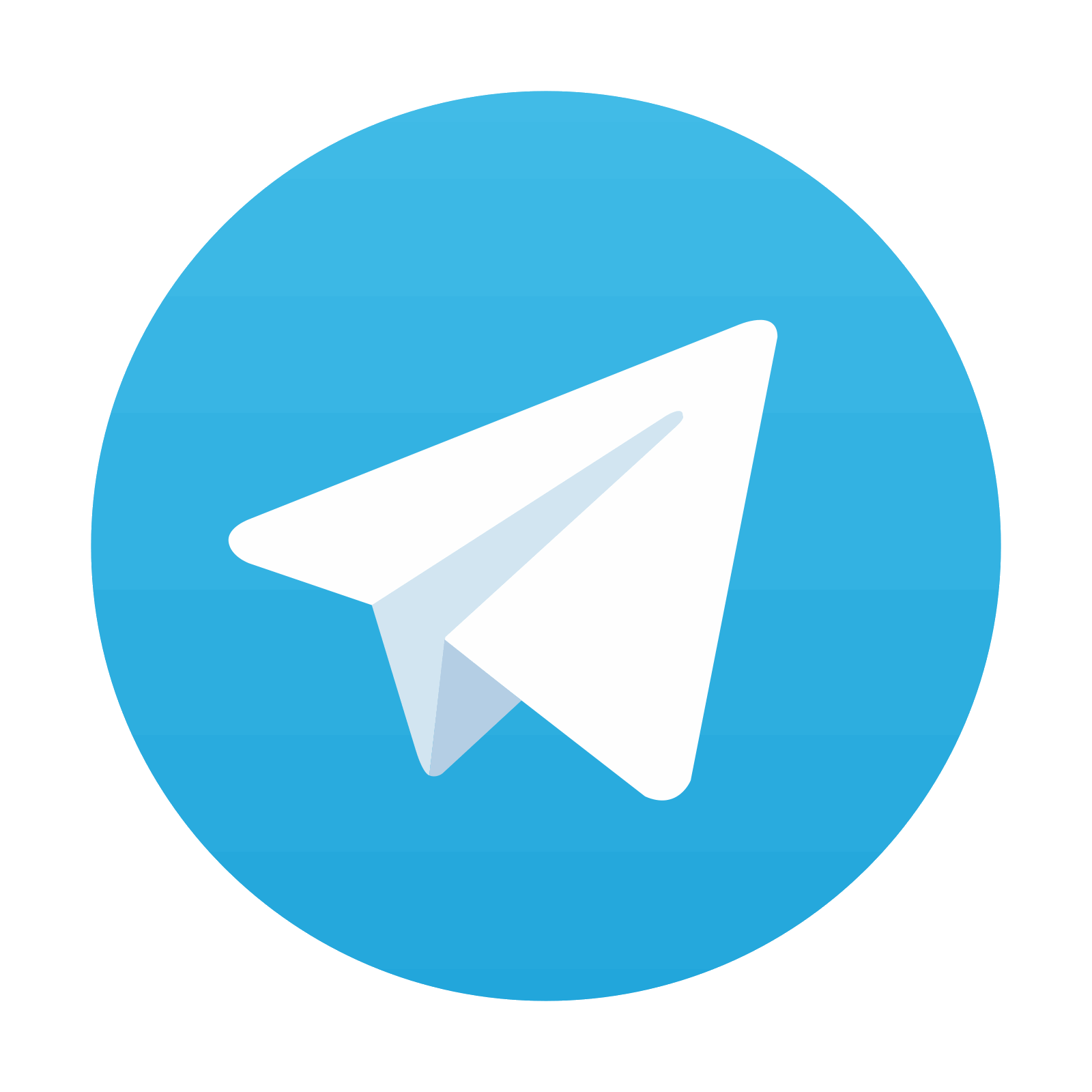
Stay updated, free articles. Join our Telegram channel
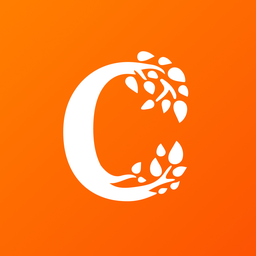
Full access? Get Clinical Tree
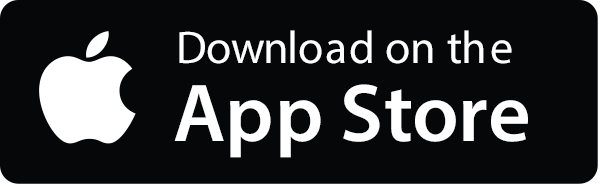
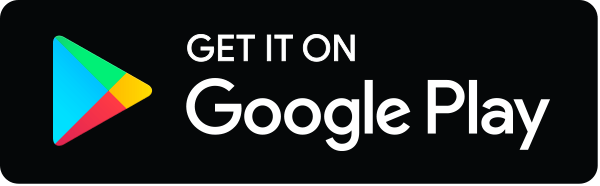
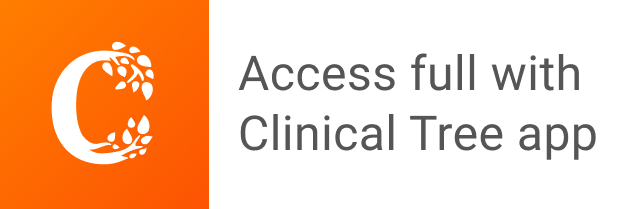