Key Features
- •
The lens comprises three parts: (1) the capsule, (2) the lens epithelium, and (3) the lens fibers.
- •
α-, β- and γ-crystallins constitute 90% of the total protein content of the lens.
- •
Lens function is dependent on the metabolism of glucose to produce energy, protein synthesis, and a complex antioxidant system.
- •
Lens transparency is dependent on the highly organized structure of the lens, the dense packing of crystallin, and the supply of appropriate nutrients.
- •
The lens can change its focusing power through a process called accommodation.
- •
The lens exhibits age-related changes in structure, light transmission, metabolic capacity, and enzyme activity.
- •
Secondary cataract occurs when residual lens cells after cataract extraction cause opacification of the visual axis.
Introduction
The lens is a vital refractive element of the human eye. The World Health Organization estimates that lens pathology (cataract) is the most common cause of blindness worldwide, affecting over 17 million people. Not surprisingly, cataract surgery is the most common procedure performed in the developed world. An understanding of the basic science of the lens provides valuable insight into the various pathologies involving the lens and their treatment.
Anatomy of the Lens
The adult human lens is an asymmetric oblate spheroid that does not possess nerves, blood vessels, or connective tissue. The lens is located behind the iris and pupil in the anterior compartment of the eye. The anterior surface is in contact with the aqueous; the posterior surface is in contact with the vitreous. The anterior pole of the lens and the front of the cornea are separated by approximately 3.5 mm. The lens is held in place by the zonular fibers (suspensory ligaments), which run between the lens and the ciliary body. These fibers, which originate in the region of the ciliary epithelium, are fibrillin rich and converge in a circular zone on the lens. Both an anterior and a posterior sheet meet the capsule 1–2 mm from the equator and are embedded into the outer part of the capsule (1–2 µm deep). It also is thought that a series of fibers meets the capsule at the equator.
Histologically the lens consists of three major components—capsule, epithelium, and lens substance ( Fig. 5.1.1 ).

Capsule
The lens is ensheathed by an elastic envelope composed of epithelial cells and fibers that allows the passage of molecules both into and out of the lens. Capsule thickness varies by location ( Fig. 5.1.2 ) and, except for the posterior capsule, increases with age. The capsule is composed of a number of lamellae stacked on top of each other that are narrowest near the outside of the capsule and widest near the cell mass. Major structural proteins and a small amount of fibronectin are found within the lamellae. This structure is continuously synthesized, anteriorly by the lens epithelium and posteriorly by the fiber cells.

Epithelial Cells
The lens epithelium is a single layer of cuboidal cells approximately 10 µm high and 15 µm wide beneath the anterior capsule that extends to the equatorial lens bow. Their basal surface adheres to the capsule, whereas their anterior surface abuts the newly formed elongating lens cytoplasm (“fibers”). Lens epithelial cells have a large array of organelles and contain dense bodies and glycogen particles. Lateral attachment to adjacent cells occurs through both desmosomes and tight junctions.
Lens epithelial cells contain three cytoskeletal elements: microfilaments (actin), intermediate filaments (vimentin), and microtubules (tubulin). These elements form a network that provides structural support, control of cell shape and volume, intracellular compartmentalization and movement of organelles, cell movement, distribution of mechanical stress, and mediation of chromosome movement during cell division.
Epithelial cell density is greatest in the central zone, where cells normally do not proliferate. These cells are the largest epithelial cells found in the lens. The proliferative capacity of epithelial cells is greatest at the equator (see Fig. 5.1.1 ). Cells here are dividing constantly, with newly formed cells being forced into the transitional zone where they elongate and differentiate to form the fiber mass of the lens.
Lens Substance
The lens substance, the bulk of the lens, is composed of densely packed lens cells with very little extracellular space. The adult lens substance consists of the nucleus and the cortex, which are often histologically indistinct. Although the size of these two regions is age dependent, a study of lenses with an average age of 61 years indicated that the nucleus accounted for approximately 84% of the diameter and thickness of the lens and the cortex for the remaining 16%. The nucleus is subdivided into embryonic, fetal, infantile, and adult nuclei (see Fig. 5.1.1 ). The embryonic nucleus contains the original primary lens fiber cells that are formed in the lens vesicle. The rest of the nuclei are composed of secondary fibers, which are added concentrically at the different stages of growth by encircling the previously formed nucleus. The cortex, which is located peripherally, is composed of all the secondary fibers formed after sexual maturation.
Fibers are formed constantly throughout life by the elongation of lens epithelial cells at the equator. Initially, transitional columnar cells are formed, but once long enough, the anterior end moves forward beneath the anterior epithelial cell layer and the posterior end is pushed backward along the posterior capsule. The ends of this U-shaped fiber run toward the poles of both capsular surfaces. Once fully matured, the fiber detaches from the anterior epithelium and the posterior capsule. Each new layer of secondary fibers formed at the periphery of the lens constitutes a new growth shell. Lens fibers are bound by the interlocking of the lateral plasma membranes of adjacent fibers. Both desmosomes and tight junctions are absent from mature lens fibers, although desmosomes are found between elongating fibers.
Sutures
Sutures are found at both the anterior and the posterior poles. They are formed by the overlap of ends of secondary fibers in each growth shell. No sutures are found between the primary fibers in the embryonic nucleus. Each growth shell of secondary fibers formed before birth has an anterior suture shaped as an “erect Y” and a posterior suture shaped as an inverted Y. The formation of sutures enables the shape of the lens to change from spherical to a flattened biconvex sphere.
Growth
The lens continuously grows throughout life but at a reduced rate with increased age. The number of both epithelial cells and fibers increases by approximately 45%–50% during the first two decades of life ( Fig. 5.1.3 ). After this, the increase in cell numbers is reduced, with the proportional increase in fibers being very small. During an average lifespan, the surface area of the lens capsule increases from 80 mm 2 at birth to 180 mm 2 by the seventh decade.

Mass
The weight of the lens increases rapidly from 65 mg at birth to 125 mg by the end of the first year. It then increases at approximately 2.8 mg/year until the end of the first decade, reaching 150 mg. Thereafter, the rate slows to reach a weight of 260 mg by the age of 90 (see Fig. 5.1.3 ). The average male lens weighs more than that of an age-matched female, with a mean difference of 7.9 ± 2.47 mg.
Dimensions
The equatorial diameter of the human lens increases throughout life, slowing after the second decade. The diameter grows from approximately 5 mm at birth to 9–10 mm in a 20-year-old. The thickness of the lens also increases but at a much slower rate. The distance from the anterior to the posterior poles grows from 3.5–4 mm at birth, to 4.75–5 mm (unaccommodated). The thickness of the nucleus decreases with age due to compaction, whereas cortical thickness increases as more fibers are added at the periphery. Because the increase in cortical thickness is greater than the decrease in size of the nucleus, the polar axis of the lens increases with age. The radius of curvature of the anterior surface decreases from 16 mm at the age of 10 years to 8 mm by the age of 80 years. There is very little change in the radius of curvature of the posterior surface, which remains at approximately 8 mm.
Capsule
The lens is ensheathed by an elastic envelope composed of epithelial cells and fibers that allows the passage of molecules both into and out of the lens. Capsule thickness varies by location ( Fig. 5.1.2 ) and, except for the posterior capsule, increases with age. The capsule is composed of a number of lamellae stacked on top of each other that are narrowest near the outside of the capsule and widest near the cell mass. Major structural proteins and a small amount of fibronectin are found within the lamellae. This structure is continuously synthesized, anteriorly by the lens epithelium and posteriorly by the fiber cells.

Epithelial Cells
The lens epithelium is a single layer of cuboidal cells approximately 10 µm high and 15 µm wide beneath the anterior capsule that extends to the equatorial lens bow. Their basal surface adheres to the capsule, whereas their anterior surface abuts the newly formed elongating lens cytoplasm (“fibers”). Lens epithelial cells have a large array of organelles and contain dense bodies and glycogen particles. Lateral attachment to adjacent cells occurs through both desmosomes and tight junctions.
Lens epithelial cells contain three cytoskeletal elements: microfilaments (actin), intermediate filaments (vimentin), and microtubules (tubulin). These elements form a network that provides structural support, control of cell shape and volume, intracellular compartmentalization and movement of organelles, cell movement, distribution of mechanical stress, and mediation of chromosome movement during cell division.
Epithelial cell density is greatest in the central zone, where cells normally do not proliferate. These cells are the largest epithelial cells found in the lens. The proliferative capacity of epithelial cells is greatest at the equator (see Fig. 5.1.1 ). Cells here are dividing constantly, with newly formed cells being forced into the transitional zone where they elongate and differentiate to form the fiber mass of the lens.
Lens Substance
The lens substance, the bulk of the lens, is composed of densely packed lens cells with very little extracellular space. The adult lens substance consists of the nucleus and the cortex, which are often histologically indistinct. Although the size of these two regions is age dependent, a study of lenses with an average age of 61 years indicated that the nucleus accounted for approximately 84% of the diameter and thickness of the lens and the cortex for the remaining 16%. The nucleus is subdivided into embryonic, fetal, infantile, and adult nuclei (see Fig. 5.1.1 ). The embryonic nucleus contains the original primary lens fiber cells that are formed in the lens vesicle. The rest of the nuclei are composed of secondary fibers, which are added concentrically at the different stages of growth by encircling the previously formed nucleus. The cortex, which is located peripherally, is composed of all the secondary fibers formed after sexual maturation.
Fibers are formed constantly throughout life by the elongation of lens epithelial cells at the equator. Initially, transitional columnar cells are formed, but once long enough, the anterior end moves forward beneath the anterior epithelial cell layer and the posterior end is pushed backward along the posterior capsule. The ends of this U-shaped fiber run toward the poles of both capsular surfaces. Once fully matured, the fiber detaches from the anterior epithelium and the posterior capsule. Each new layer of secondary fibers formed at the periphery of the lens constitutes a new growth shell. Lens fibers are bound by the interlocking of the lateral plasma membranes of adjacent fibers. Both desmosomes and tight junctions are absent from mature lens fibers, although desmosomes are found between elongating fibers.
Sutures
Sutures are found at both the anterior and the posterior poles. They are formed by the overlap of ends of secondary fibers in each growth shell. No sutures are found between the primary fibers in the embryonic nucleus. Each growth shell of secondary fibers formed before birth has an anterior suture shaped as an “erect Y” and a posterior suture shaped as an inverted Y. The formation of sutures enables the shape of the lens to change from spherical to a flattened biconvex sphere.
Growth
The lens continuously grows throughout life but at a reduced rate with increased age. The number of both epithelial cells and fibers increases by approximately 45%–50% during the first two decades of life ( Fig. 5.1.3 ). After this, the increase in cell numbers is reduced, with the proportional increase in fibers being very small. During an average lifespan, the surface area of the lens capsule increases from 80 mm 2 at birth to 180 mm 2 by the seventh decade.

Mass
The weight of the lens increases rapidly from 65 mg at birth to 125 mg by the end of the first year. It then increases at approximately 2.8 mg/year until the end of the first decade, reaching 150 mg. Thereafter, the rate slows to reach a weight of 260 mg by the age of 90 (see Fig. 5.1.3 ). The average male lens weighs more than that of an age-matched female, with a mean difference of 7.9 ± 2.47 mg.
Dimensions
The equatorial diameter of the human lens increases throughout life, slowing after the second decade. The diameter grows from approximately 5 mm at birth to 9–10 mm in a 20-year-old. The thickness of the lens also increases but at a much slower rate. The distance from the anterior to the posterior poles grows from 3.5–4 mm at birth, to 4.75–5 mm (unaccommodated). The thickness of the nucleus decreases with age due to compaction, whereas cortical thickness increases as more fibers are added at the periphery. Because the increase in cortical thickness is greater than the decrease in size of the nucleus, the polar axis of the lens increases with age. The radius of curvature of the anterior surface decreases from 16 mm at the age of 10 years to 8 mm by the age of 80 years. There is very little change in the radius of curvature of the posterior surface, which remains at approximately 8 mm.
Physiology of the Lens
Permeability, Diffusion, and Transport
After involution of the hyaloid blood supply to the lens, its metabolic needs are met by the aqueous and vitreous humor. The capsule is freely permeable to water, ions, other small molecules, and proteins with a molecular weight of up to 70 kDa. Epithelial cells and fibers possess a number of channels, pumps, and transporters that enable transepithelial movement to and from the extracellular milieu.
Transport of Ions
Fiber cells contain large concentrations of negatively charged crystallins. As a result, positively charged cations enter the lens cell to maintain electrical neutrality, and the osmolarity of the intracellular fluid becomes greater than that of the extracellular fluid. Fluid flow and swelling are minimized by the resting potential of the plasma membrane being set at a negative voltage through potassium (K + )-selective channels.
The Na + ions that leak into the cells are exchanged actively for K + ions, which diffuse through the lens down their concentration gradient and leave through ion channels in both the epithelial cells and surface fibers. There is a net movement of Na + ions from posterior to anterior and of K + ions from anterior to posterior.
Although a pH gradient exists, which increases from the central nucleus to the periphery, the intracellular pH of the lens is approximately 7.0. Lens cells need to continually extrude intracellular protons, which accumulate due to inward movement of positive ions from the extracellular space and lactic acid from anaerobic glycolysis. The pH is regulated by mechanisms capable of increasing and decreasing intracellular acid levels. Molecules, especially proteins, also act as buffers.
Amino Acid and Sugar Transport
The majority of amino acids and glucose enter the lens from the aqueous across its anterior surface. In addition, the lens can convert keto acids into amino acids. The lens acts as a pump–leak system: Amino acids are “pumped” into the lens through the anterior capsule and passively “leak” out through the posterior capsule.
Permeability, Diffusion, and Transport
After involution of the hyaloid blood supply to the lens, its metabolic needs are met by the aqueous and vitreous humor. The capsule is freely permeable to water, ions, other small molecules, and proteins with a molecular weight of up to 70 kDa. Epithelial cells and fibers possess a number of channels, pumps, and transporters that enable transepithelial movement to and from the extracellular milieu.
Transport of Ions
Fiber cells contain large concentrations of negatively charged crystallins. As a result, positively charged cations enter the lens cell to maintain electrical neutrality, and the osmolarity of the intracellular fluid becomes greater than that of the extracellular fluid. Fluid flow and swelling are minimized by the resting potential of the plasma membrane being set at a negative voltage through potassium (K + )-selective channels.
The Na + ions that leak into the cells are exchanged actively for K + ions, which diffuse through the lens down their concentration gradient and leave through ion channels in both the epithelial cells and surface fibers. There is a net movement of Na + ions from posterior to anterior and of K + ions from anterior to posterior.
Although a pH gradient exists, which increases from the central nucleus to the periphery, the intracellular pH of the lens is approximately 7.0. Lens cells need to continually extrude intracellular protons, which accumulate due to inward movement of positive ions from the extracellular space and lactic acid from anaerobic glycolysis. The pH is regulated by mechanisms capable of increasing and decreasing intracellular acid levels. Molecules, especially proteins, also act as buffers.
Amino Acid and Sugar Transport
The majority of amino acids and glucose enter the lens from the aqueous across its anterior surface. In addition, the lens can convert keto acids into amino acids. The lens acts as a pump–leak system: Amino acids are “pumped” into the lens through the anterior capsule and passively “leak” out through the posterior capsule.
Transport of Ions
Fiber cells contain large concentrations of negatively charged crystallins. As a result, positively charged cations enter the lens cell to maintain electrical neutrality, and the osmolarity of the intracellular fluid becomes greater than that of the extracellular fluid. Fluid flow and swelling are minimized by the resting potential of the plasma membrane being set at a negative voltage through potassium (K + )-selective channels.
The Na + ions that leak into the cells are exchanged actively for K + ions, which diffuse through the lens down their concentration gradient and leave through ion channels in both the epithelial cells and surface fibers. There is a net movement of Na + ions from posterior to anterior and of K + ions from anterior to posterior.
Although a pH gradient exists, which increases from the central nucleus to the periphery, the intracellular pH of the lens is approximately 7.0. Lens cells need to continually extrude intracellular protons, which accumulate due to inward movement of positive ions from the extracellular space and lactic acid from anaerobic glycolysis. The pH is regulated by mechanisms capable of increasing and decreasing intracellular acid levels. Molecules, especially proteins, also act as buffers.
Amino Acid and Sugar Transport
The majority of amino acids and glucose enter the lens from the aqueous across its anterior surface. In addition, the lens can convert keto acids into amino acids. The lens acts as a pump–leak system: Amino acids are “pumped” into the lens through the anterior capsule and passively “leak” out through the posterior capsule.
Biophysics
Light Transmission
The lens acts as a spectral filter absorbing long ultraviolet B (UV-B, 300–315 nm) and most of the UV-A (315–400 nm) wavelengths. While there is a transmission band centered around 320 nm of about 8% in children under 10 years, it is reduced to 0.1% by age 22. By age 60, no UV radiation transmits across the lens. The total transmittance of the young lens begins increasing rapidly at about 310 nm and reaches 90% at 450 nm, compared with the older lens, which begins transmitting at 400 nm but does not reach 90% total transmittance until 540 nm ( Fig. 5.1.4 ). The overall transmission of visible light decreases with increasing age, a feature that arises largely from age-related changes and brunescence in the lens (see Fig. 5.1.4 ).

Transparency
The lens is opaque during the early stages of embryonic development. As development continues and the hyaloid vascular supply is lost, the lens becomes transparent. Transparency is due to the absence of chromophores able to absorb visible light and the presence of a uniform structure that scatters light minimally (less than 5% in the normal human lens). Light scatter is minimized in fiber cells once the fibers have elongated and their organelles have degenerated. Although the epithelial cells contain large organelles that scatter light, the combined refractive index of this layer and the capsule is no different from the refractive index of the aqueous, so light scatter is very small.
Refractive Indices
The refractive index increases from 1.386 in the peripheral cortex to 1.41 in the central nucleus of the lens. Because both the curvature and refractive index of the lens increase from the periphery toward the center, each successive layer of fibers has more refractive power and therefore can bend light rays to a greater extent. The anterior capsular surface of the lens has a greater refractive index than the posterior capsular surface (1.364–1.381 compared with 1.338–1.357). The increase in refractive index from the surface to the center results from changes in protein concentration; the higher the concentration, the greater the refractive power. This increase must occur as a result of both packing and hydration properties, because protein synthesis in the nucleus is minimal.
Chromatic Aberration
When visible light passes through the lens, it is split into all the colors of the spectrum. The different wavelengths of these colors result in different rates of transmission through the lens and some deviation. As a consequence, yellow light (570–595 nm) is normally focused on the retina; light of shorter wavelengths, for example blue (440–500 nm), falls in front because of its slower transmission and increased refraction compared with yellow light. Light of longer wavelengths, for example red (620–770 nm), falls behind because of the faster transmission and less refraction ( Fig. 5.1.5 ). Because the amount of dispersion between the red and the blue images is approximately 1.50–2.00 diopters (D), very little reduction occurs in the clarity of the image that is formed. As the lens accommodates, refraction increases as a result of the increasing power of the lens and, therefore, the amount of chromatic aberration also increases.

Spherical Aberration
The lens of the human eye is designed to minimize spherical aberration since: (1) refractive index increases from the periphery to the center of the lens; (2) curvature of both the anterior and the posterior capsule increases towards the poles; and (3) curvature of the anterior capsule is greater than that of its posterior counterpart.
As a result of these structural features, the focal points of the peripheral and central rays are similar, which ensures that reduction in the quality of the image is minimal (see Fig. 5.1.5 ). The pupil diameter also affects the amount of spherical aberration, because light rays do not pass through the periphery of the lens (unless the pupil is dilated). The optimal size of the pupil needed to minimize this imperfection is 2–2.5 mm.
Accommodation
The lens is able to change its shape and thus the focusing power of the eye. This process is known as accommodation, and it enables both distant and close objects to be brought into focus on the retina. At rest, the ciliary muscle is relaxed and the zonules pull on the lens keeping the capsule under tension and the lens flattened. Light rays from close objects are divergent and are focused behind the retina in this configuration. The lens accommodates these objects by contraction of the ciliary muscles, relaxing the zonules, thus increasing the curvature of the anterior surface and decreasing the radius of curvature from 10 mm to 6 mm. The increase in curvature of the anterior surface increases the refractive power, so that the light rays from close objects are refracted toward each other to a greater extent and, therefore, converge on the fovea. Because the front of the lens has moved forward, the depth of the anterior chamber decreases from 3.5 mm to 3.2–3.3 mm. Very little change occurs in the curvature of the posterior capsule, which remains at approximately 6 mm ( Fig. 5.1.6 ). Accommodation is accompanied by a decrease in pupil size and convergence of the two eyes.


Accommodation can be divided into both physical and physiological processes. Physical accommodation, a measure of the change in shape of the lens, is measured in terms of the amplitude of accommodation using the unit diopter. It represents a measure of the extent to which objects close to the eye can be brought into focus. Physiological accommodation, a measure of the force of ciliary muscle contraction per diopter, is measured with the unit myodiopter. The myodiopter increases during the act of accommodation.
Light Transmission
The lens acts as a spectral filter absorbing long ultraviolet B (UV-B, 300–315 nm) and most of the UV-A (315–400 nm) wavelengths. While there is a transmission band centered around 320 nm of about 8% in children under 10 years, it is reduced to 0.1% by age 22. By age 60, no UV radiation transmits across the lens. The total transmittance of the young lens begins increasing rapidly at about 310 nm and reaches 90% at 450 nm, compared with the older lens, which begins transmitting at 400 nm but does not reach 90% total transmittance until 540 nm ( Fig. 5.1.4 ). The overall transmission of visible light decreases with increasing age, a feature that arises largely from age-related changes and brunescence in the lens (see Fig. 5.1.4 ).

Transparency
The lens is opaque during the early stages of embryonic development. As development continues and the hyaloid vascular supply is lost, the lens becomes transparent. Transparency is due to the absence of chromophores able to absorb visible light and the presence of a uniform structure that scatters light minimally (less than 5% in the normal human lens). Light scatter is minimized in fiber cells once the fibers have elongated and their organelles have degenerated. Although the epithelial cells contain large organelles that scatter light, the combined refractive index of this layer and the capsule is no different from the refractive index of the aqueous, so light scatter is very small.
Refractive Indices
The refractive index increases from 1.386 in the peripheral cortex to 1.41 in the central nucleus of the lens. Because both the curvature and refractive index of the lens increase from the periphery toward the center, each successive layer of fibers has more refractive power and therefore can bend light rays to a greater extent. The anterior capsular surface of the lens has a greater refractive index than the posterior capsular surface (1.364–1.381 compared with 1.338–1.357). The increase in refractive index from the surface to the center results from changes in protein concentration; the higher the concentration, the greater the refractive power. This increase must occur as a result of both packing and hydration properties, because protein synthesis in the nucleus is minimal.
Chromatic Aberration
When visible light passes through the lens, it is split into all the colors of the spectrum. The different wavelengths of these colors result in different rates of transmission through the lens and some deviation. As a consequence, yellow light (570–595 nm) is normally focused on the retina; light of shorter wavelengths, for example blue (440–500 nm), falls in front because of its slower transmission and increased refraction compared with yellow light. Light of longer wavelengths, for example red (620–770 nm), falls behind because of the faster transmission and less refraction ( Fig. 5.1.5 ). Because the amount of dispersion between the red and the blue images is approximately 1.50–2.00 diopters (D), very little reduction occurs in the clarity of the image that is formed. As the lens accommodates, refraction increases as a result of the increasing power of the lens and, therefore, the amount of chromatic aberration also increases.

Spherical Aberration
The lens of the human eye is designed to minimize spherical aberration since: (1) refractive index increases from the periphery to the center of the lens; (2) curvature of both the anterior and the posterior capsule increases towards the poles; and (3) curvature of the anterior capsule is greater than that of its posterior counterpart.
As a result of these structural features, the focal points of the peripheral and central rays are similar, which ensures that reduction in the quality of the image is minimal (see Fig. 5.1.5 ). The pupil diameter also affects the amount of spherical aberration, because light rays do not pass through the periphery of the lens (unless the pupil is dilated). The optimal size of the pupil needed to minimize this imperfection is 2–2.5 mm.
Accommodation
The lens is able to change its shape and thus the focusing power of the eye. This process is known as accommodation, and it enables both distant and close objects to be brought into focus on the retina. At rest, the ciliary muscle is relaxed and the zonules pull on the lens keeping the capsule under tension and the lens flattened. Light rays from close objects are divergent and are focused behind the retina in this configuration. The lens accommodates these objects by contraction of the ciliary muscles, relaxing the zonules, thus increasing the curvature of the anterior surface and decreasing the radius of curvature from 10 mm to 6 mm. The increase in curvature of the anterior surface increases the refractive power, so that the light rays from close objects are refracted toward each other to a greater extent and, therefore, converge on the fovea. Because the front of the lens has moved forward, the depth of the anterior chamber decreases from 3.5 mm to 3.2–3.3 mm. Very little change occurs in the curvature of the posterior capsule, which remains at approximately 6 mm ( Fig. 5.1.6 ). Accommodation is accompanied by a decrease in pupil size and convergence of the two eyes.


Accommodation can be divided into both physical and physiological processes. Physical accommodation, a measure of the change in shape of the lens, is measured in terms of the amplitude of accommodation using the unit diopter. It represents a measure of the extent to which objects close to the eye can be brought into focus. Physiological accommodation, a measure of the force of ciliary muscle contraction per diopter, is measured with the unit myodiopter. The myodiopter increases during the act of accommodation.
Biochemistry
The lens requires energy to drive thermodynamically unfavorable reactions. Adenosine triphosphate (ATP) is the principal source of this energy, the majority of which comes from the anaerobic metabolism of glucose. Nicotinamide adenine dinucleotide phosphate (NADPH), which is produced principally via the pentose phosphate pathway, is used as a reducing agent in the biosynthesis of many essential cellular components, such as fatty acids and glutathione.
Sugar Metabolism
Approximately 90%–95% of the glucose that enters the normal lens is phosphorylated into glucose-6-phosphate (G6P) in a reaction catalyzed by hexokinase. Although this enzyme exists as three different isoforms, only types I and II have been found in the lens. Type I has a greater affinity for glucose and is concentrated in the nucleus, where glucose levels are low. Type II, which accounts for 70% of the hexokinase, has a lower affinity for glucose and is found predominantly in the epithelium and cortex, where glucose levels are higher. G6P is used either in the glycolytic pathway (80% of total glucose) or in the pentose phosphate pathway (hexose monophosphate shunt; 10% of total glucose) ( Fig. 5.1.7 ). Hexokinase is saturated by physiological concentrations of glucose found in the lens and limits the rate of both glycolysis and the pentose phosphate pathway. Glycolysis also is regulated by phosphofructokinase and pyruvate kinase.

The lens lacks vascularity and thus exists in a hypoxic environment, which results in at least 70% of lens ATP being derived from anaerobic glycolysis. Approximately 3% of lens glucose passes into the more efficient tricarboxylic acid cycle (see Fig. 5.1.7 ), generating 25% of lens ATP. Glycolysis and the tricarboxylic acid cycle generate two energy-rich molecules; the reduced form of nicotinamide adenine dinucleotide (NADH) and the reduced form of flavin adenine dinucleotide (FADH2). These donate their electrons to oxygen, which releases large amounts of free energy that is subsequently used to generate ATP. This cycle, which is restricted to the epithelial layer, also provides carbon skeleton intermediates for biosynthesis, such as amino acids and porphyrins.
The bulk of the pyruvate produced by the glycolytic pathway is reduced to lactate by lactate dehydrogenase (see Fig. 5.1.7 ), which is concentrated in the cortex. The formation of lactate results in the reoxidation of NADH to NAD + . Glyceraldehyde-3-phosphate dehydrogenase regulates the activity of lactate dehydrogenase by controlling the rate of conversion of glyceraldehyde-3-phosphate into 1,3-diphosphoglycerate and, therefore, the availability of NADH.
The 5%–10% of glucose that is not phosphorylated into G6P either enters the sorbitol pathway or is converted into gluconic acid (see Fig. 5.1.7 ). Glucose is converted into sorbitol by aldose reductase, an enzyme localized to the epithelial layer. Sorbitol is converted by polyol dehydrogenase into fructose, a less optimal substrate for glycolysis. Both sorbitol and fructose have the potential to increase osmotic pressure and may help to regulate the volume of the lens.
Protein Metabolism
The protein concentration within the lens is the highest in the body. The majority of ongoing synthesis creates crystallins and major intrinsic protein 26 (MIP26). It is thought that this occurs in the epithelial cells and cortical fibers, which contain the organelles needed.
Lens proteins remain stable for long periods because the majority of the degradative enzymes normally are inhibited. This is coordinated by marking those to be degraded with a small 8.5 kDa protein called ubiquitin. This system, which is ATP dependent, is most active in the epithelial layer. Lens proteins are broken down into peptides by endopeptidases and then into amino acids by exopeptidases. Neutral endopeptidase is activated by both calcium and magnesium and is optimal at pH 7.5 (the pH of the lens is approximately 7.0–7.2). The principal substrate of this enzyme is α-crystallin. The calpains (I and II) are localized mainly in the epithelial cells and cortex and function to degrade crystallins and cytoskeletal proteins. They are cysteine endopeptidases, the activities of which are regulated by calcium. These enzymes are inhibited by calpastatin, a natural inhibitor found at higher concentrations than the calpains. The lens also contains a serine proteinase and a membrane-bound proteinase. The main exopeptidase is leucine aminopeptidase, an enzyme optimal at pH 8.5–9.0, which catalyzes the removal of amino acids from the N-terminal of peptides. Aminopeptidase III has an optimal pH of 6.0 and as a result has a greater activity than leucine aminopeptidase in the normal lens.
Glutathione
Glutathione is found at high concentrations in the lens (3.5–5.5 mmol/g wet weight), especially in the epithelial layer. Glutathione has many important roles in the lens, including :
- •
Maintaining protein thiols in the reduced state, which helps to maintain lens transparency by preventing the formation of high molecular weight crystallin aggregates.
- •
Protection of thiol groups involved in cation transport and permeability; for example, oxidation of the -SH groups of the Na + ,K + -ATPase pump, which results in an increased permeability to these ions.
- •
Protection against oxidative damage (see later in this chapter).
- •
Removal of xenobiotics; glutathione-S-transferase catalyzes the conjugation of glutathione to hydrophobic compounds with an electrophilic center.
Amino Acid Transport
Glutathione has a half-life of 1–2 days and is recycled constantly by the γ-glutamyl cycle; its synthesis and degradation occur at approximately the same rate ( Fig. 5.1.8 ). Glutathione is synthesized from L-glutamate, L-cysteine, and glycine in a two-step process that uses 11%–12% of lens ATP. Reduced glutathione also can be taken into the lens from the aqueous. A reduced glutathione transporter that allows the uptake of glutathione by the lens epithelium has been characterized. The breakdown of glutathione releases its amino acids, which are recycled.

Antioxidant Mechanisms
The term reactive oxygen species (ROS) refers to highly reactive oxygen radicals that have the potential to damage lipids, proteins, carbohydrates, and nucleic acids. These include the superoxide anion, the hydroxyl free radical, hydroperoxyl radicals, lipid peroxyl radicals, singlet oxygen, and hydrogen peroxide (H 2 O 2 ). ROS generally arise from cell metabolism or photochemical reactions. Photochemical damage occurs when light is absorbed by a photosensitizer that, upon photoexcitation, forms a transient excited triplet state that is long lived, allowing for interaction with other molecules producing free radicals or singlet oxygen. The continuous entry of optical radiation into the lens, in particular the absorption of shorter wavelengths (295–400 nm), makes lens tissue particularly susceptible to photochemical reactions. The major ultraviolet (UV) absorbers in the lens are free or bound aromatic amino acids (e.g., tryptophan), numerous pigments (e.g., 3-hydroxykynurenine), and fluorophores. Reactive oxygen species also can enter the lens from the surrounding milieu (e.g., H 2 O 2 is present at high levels in the aqueous humor, 30 mmol/L in humans).
ROS have the capacity to damage the lens in many ways :
- •
Peroxidizing membrane lipids results in the formation of aldehydes, which in turn can form cross-links between membrane lipids and proteins.
- •
Introducing damage into the bases of the DNA (e.g., base modifications) and reducing DNA repair efficiency.
- •
Polymerizing and crosslinking proteins result in crystallin aggregation and inactivation of many essential enzymes, including those with an antioxidant role (e.g., catalase and glutathione reductase).
Protection against damage induced by ROS is achieved in a number of ways. The superoxide anion undergoes dismutation by superoxide dismutase or by interaction with ascorbate (see below), which results in the formation of H 2 O 2 . This, along with the high levels of exogenous H 2 O 2 , is detoxified by the enzyme catalase or glutathione peroxidase or both ( Fig. 5.1.9 ). Catalase is present in epithelial cells at higher levels than in fibers. Glutathione peroxidase, however, is found in significant amounts in both epithelial cells and fibers. The glutathione system is thought to provide the most protection against H 2 O 2, but it also protects against the lipid-free radical chain reaction by the neutralization of lipid peroxides.

Ascorbic acid (vitamin C) plays a major role in the antioxidant system, although this may be species dependent, because the human lens is rich in ascorbate (1.9 mg/kg wet weight or 1.1 mmol/kg). Ascorbate is present at high levels in the outer layers of the lens but virtually absent from the nucleus. It reacts rapidly with superoxide anions, peroxide radicals, and hydroxyl radicals to give dehydroascorbate. It also scavenges singlet oxygen, reduces thiol radicals, and is important in the prevention of lipid peroxidation. The ascorbic acid and glutathione systems are coupled, as dehydroascorbate reacts with the reduced form of glutathione to generate ascorbate and GSSG (oxidized glutathione).
This system, however, is not 100% efficient, and a low level of cumulative damage occurs throughout life.
Sugar Metabolism
Approximately 90%–95% of the glucose that enters the normal lens is phosphorylated into glucose-6-phosphate (G6P) in a reaction catalyzed by hexokinase. Although this enzyme exists as three different isoforms, only types I and II have been found in the lens. Type I has a greater affinity for glucose and is concentrated in the nucleus, where glucose levels are low. Type II, which accounts for 70% of the hexokinase, has a lower affinity for glucose and is found predominantly in the epithelium and cortex, where glucose levels are higher. G6P is used either in the glycolytic pathway (80% of total glucose) or in the pentose phosphate pathway (hexose monophosphate shunt; 10% of total glucose) ( Fig. 5.1.7 ). Hexokinase is saturated by physiological concentrations of glucose found in the lens and limits the rate of both glycolysis and the pentose phosphate pathway. Glycolysis also is regulated by phosphofructokinase and pyruvate kinase.

The lens lacks vascularity and thus exists in a hypoxic environment, which results in at least 70% of lens ATP being derived from anaerobic glycolysis. Approximately 3% of lens glucose passes into the more efficient tricarboxylic acid cycle (see Fig. 5.1.7 ), generating 25% of lens ATP. Glycolysis and the tricarboxylic acid cycle generate two energy-rich molecules; the reduced form of nicotinamide adenine dinucleotide (NADH) and the reduced form of flavin adenine dinucleotide (FADH2). These donate their electrons to oxygen, which releases large amounts of free energy that is subsequently used to generate ATP. This cycle, which is restricted to the epithelial layer, also provides carbon skeleton intermediates for biosynthesis, such as amino acids and porphyrins.
The bulk of the pyruvate produced by the glycolytic pathway is reduced to lactate by lactate dehydrogenase (see Fig. 5.1.7 ), which is concentrated in the cortex. The formation of lactate results in the reoxidation of NADH to NAD + . Glyceraldehyde-3-phosphate dehydrogenase regulates the activity of lactate dehydrogenase by controlling the rate of conversion of glyceraldehyde-3-phosphate into 1,3-diphosphoglycerate and, therefore, the availability of NADH.
The 5%–10% of glucose that is not phosphorylated into G6P either enters the sorbitol pathway or is converted into gluconic acid (see Fig. 5.1.7 ). Glucose is converted into sorbitol by aldose reductase, an enzyme localized to the epithelial layer. Sorbitol is converted by polyol dehydrogenase into fructose, a less optimal substrate for glycolysis. Both sorbitol and fructose have the potential to increase osmotic pressure and may help to regulate the volume of the lens.
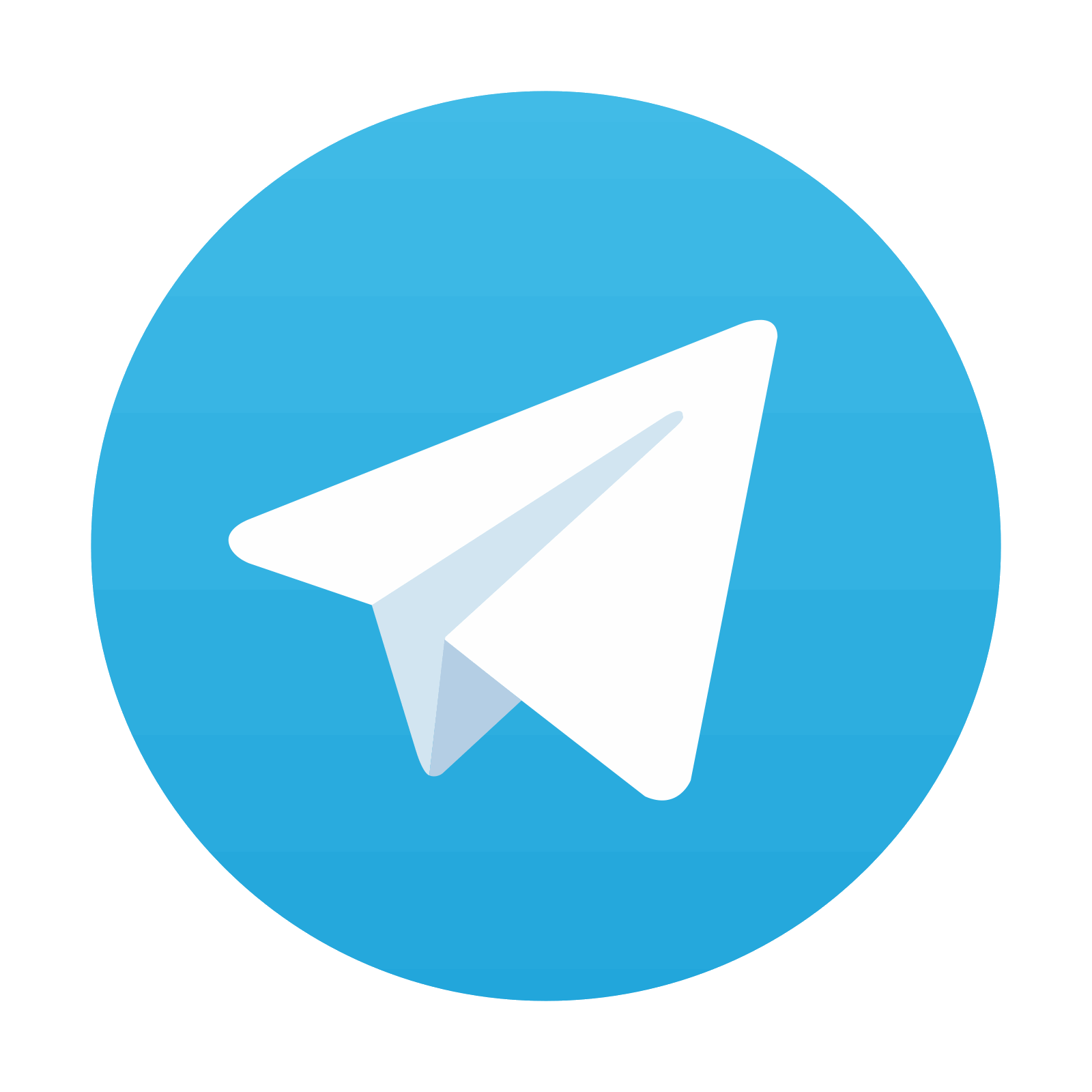
Stay updated, free articles. Join our Telegram channel
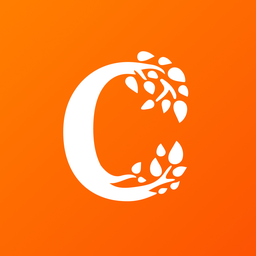
Full access? Get Clinical Tree
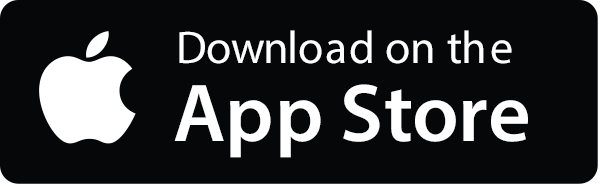
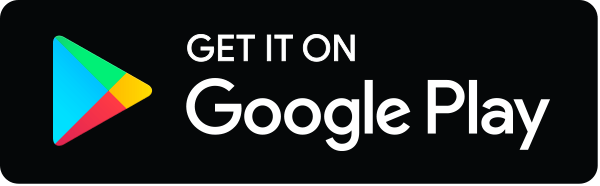