Introduction
“There is no other portion of physiological optics where one finds so many differing and contradictory ideas as concerns the accommodation of the eye where only recently in the most recent time have we actually made observations where previously everything was left to the play of hypotheses”
H Von Helmholtz (1909)
It is primarily due to Helmholtz that we owe our current understanding of the accommodative mechanism of the human eye ( Fig. 3.1 ). His insight came from his own work and from pioneers before him. Thomas Young was instrumental in demonstrating that accommodation occurs, not through changes in corneal curvature or axial length as those before him believed, but through changes in the curvature of the lens. Young’s painstaking anatomical investigations were insufficient for him to rule out the possibility that the crystalline lens received direct innervation from a branch of the ciliary nerves to allow it to contract as a muscle. It was only after the work of Crampton, who first described the ciliary muscle from his investigation of bird eyes, that a mechanistic description of how the ciliary muscle might alter lens curvatures was proposed by Müller. Understanding of human accommodation was mired by confusion from numerous investigations of the eyes of birds and other vertebrates, studied for their comparatively large size to gain insight into the human accommodative mechanism ( Box 3.1 ). However, these species are now known to accommodate through mechanisms quite different from humans. Current understanding of accommodation stems from the work of many early investigators including Brücke, Cramer, Hess, Müller, Helmholtz and Gullstrand. This path was made tortuous by the diversity of accommodative mechanisms of the various vertebrates studied. Possibly the most ancient of accommodative mechanisms is that of the sauropsidae (lizards, birds and turtles). Although these eyes differ from the primate eye, these species share many unusual ocular characteristics among themselves including striated intraocular muscles, bony plates or ossicles in the sclera, attachment of the ciliary processes to the lens equator, the absence of a circumlental space, a lens annular pad, and in some species at least, corneal accommodation and iris-mediated lenticular accommodation.

- •
Accommodation is a dioptric change in optical power of the eye due to ciliary muscle contraction
- •
Accommodation occurs largely in accordance with the mechanism originally proposed by Helmholtz
- •
Ciliary muscle contraction moves the apex of the ciliary body towards the axis of the eye and releases resting zonular tension around the lens equator
- •
When zonular tension is released, the elastic lens capsule molds the young lens into a more spherical and accommodated form
- •
During accommodation, lens diameter decreases, lens thickness increases, the anterior lens surface moves anteriorly, the posterior lens surface moves posteriorly and the lens anterior and posterior surface curvatures increase, the thickness of the nucleus increases, but without a change in thickness of the cortex
- •
The increase in curvature of the lens anterior and posterior surfaces results in an increase in optical power of the lens
- •
The physical changes in the lens and eye result in an increase in optical power of the eye to focus on near objects
The wide diversity of avian visual habitats (aerial, aquatic, terrestrial), eye shapes (tubular, globose and flattened), and feeding behaviors in all likelihood dictates their accommodative needs. Corneal accommodation, of considerable value to terrestrial birds, is of no value to aquatic birds where the corneal optical power is neutralized under water. The evolutionarily divergent accommodative mechanisms, or the absence of accommodation in other vertebrates is, by reasonable conjecture, determined by feeding behaviors. Herbivorous animals (sheep, horses, cows, etc.), those which forage and dig for food primarily using olfactory cues (pigs), or those with nocturnal eyes and relatively poor visual abilities (mice, rats, rabbits) have little need for accommodation. Carnivores have better-developed ciliary muscles than these other species, but still have relatively little accommodative ability; the raccoon is the only non-primate terrestrial mammal with substantial accommodative amplitude. Cats are suggested and raccoons and fish shown to translate the lens forward without lenticular thickening. Other adaptations in the lens, iris, or retina allow other lower vertebrates functional near and distance vision, although these cannot be classified as true accommodation since they rely on static optical adaptations.
Among the vertebrates that do accommodate, amplitudes vary considerably. Diving birds have among the largest amplitudes with cormorant having ~50 D and diving ducks suggested to have 70–80D. Among the mammals, vervet and cynomolgus monkeys have approximately 20 D, young rhesus as much as 40 D and raccoons about 20 D. Humans, for only a few short childhood years, may have a maximum of about 10–15D measured subjectively or about 7–8 D measured objectively, but find much less accommodation adequate for most visual tasks. Although accommodative amplitude gradually declines until completely lost by about age 50 years, to most individuals the deficit appears to be of sudden onset when the accommodative amplitude is diminished to a few diopters as presbyopia develops. Although presbyopes may read at intermediate distances, this is almost certainly due to depth of field (see below) resulting from pupil constriction rather than active accommodation. The word presbyopia (Greek, presbys meaning an aged person and opsis meaning vision) possibly derives from Aristotle’s use of the term presbytas to describe “those who see well at distance, but poorly at near”. Historically the term was used to describe the condition where the near point has receded too far from the eye due to a diminution in the range of accommodation. Despite the wealth of studies of accommodation on vertebrates, only primates are shown to systematically lose the ability to accommodate with increasing age. It may be no coincidence that although absolute life spans differ considerably, the relative age course of the progression of presbyopia is similar in humans and monkeys ( Fig. 3.2 ).

Accommodation
Accommodation is a dynamic, optical change in the dioptric power of the eye allowing the point of focus of the eye to be changed from distant to near objects. In primates this is mediated through a contraction of the ciliary muscle, release of resting zonular tension around the lens equator, a decrease in lens diameter and a “rounding up” of the crystalline lens through the force exerted on the lens by the lens capsule. The increased optical power of the lens is achieved through increased anterior and posterior surface curvatures and increased thickness. In an unaccommodated, emmetropic eye (an eye without refractive error) distant objects at or beyond what is considered optical infinity for the eye (6 m or 20 ft) are focused on the retina. When an object is brought closer to the eye, the eye must accommodate to maintain a clearly focused image on the retina. Myopic eyes, typically too long for the optical power of the lens and cornea combined, are unable to attain a sharply focused image for objects at optical infinity unless optical compensation is provided such as through negative powered spectacle lenses. Myopes can focus clearly on objects closer to the eye than optical infinity without accommodation (i.e. objects at their far point). Young hyperopes are only able to focus clearly on objects at optical infinity through an accommodative increase in the optical power of the eye provided their accommodative amplitude exceeds the amount of hyperopia.
Optics of the eye
Light from the environment enters the eye at the cornea and, in an emmetropic eye, is brought to a focus on the retina through the combined optical power of the cornea and the lens (see Chapter 1 ). When light from an object is focused on the retina, a clear, sharp image is perceived. This enables the performance of near visual tasks such as reading. If the image is not focused on the retina, these tasks become difficult or impossible to perform without optical compensation to bring the image to focus on the retina.
The optical elements of the eye, cornea, the aqueous humor, the crystalline lens and the vitreous humor all contribute to the optical power of the eye (see Chapters 1 & 2 ). Specific details for schematic eyes are given in Bennett & Rabbetts. In the adult human eye an average, normal cornea has a radius of curvature of about +7.8 mm, a thickness of about 0.25 mm near the optical axis and the cornea provides about 70 percent of the optical refracting power of the eye. Light passes from an air environment, with a refractive index of approximately 1.00, through the tear film and into the cornea. The cornea is composed largely of fluid and proteins and therefore has a refractive index greater than air of about 1.376. The optical power of the cornea is due to a combination of the positive radius of curvature and the higher corneal refractive index than the surrounding air. Light then passes through the cornea and into the aqueous humor. Since the refractive index of the aqueous humor is close to that of the cornea (about 1.336) there is relatively little optical effect at the posterior cornea/aqueous interface. Light then enters the anterior surface of the crystalline lens. The surface of the crystalline lens has a refractive index slightly higher than that of the aqueous humor (about 1.386). The lens anterior surface has a radius of curvature of about +11.00 mm which adds to the optical power of the eye. The crystalline lens has a gradient refractive index that progressively increases from about 1.362 at the surface of the cortex to about 1.406 at the center of the nucleus of the lens. The gradient refractive index of the lens adds additional optical power to the lens because the gradient results in refraction of light throughout the lens. This results in light taking a curved path rather than a straight path through the lens. For simplified optical calculations, the more complex gradient refractive index of the lens is often substituted with a single equivalent refractive index value.
The extent to which the gradient refractive index adds additional optical power to the lens is evident when it is realized that for an equivalent refractive index lens to have the same shape and optical power as a gradient refractive index lens, the equivalent refractive index value must be greater than the highest refractive index value at the center of the gradient refractive index lens. The posterior surface of the crystalline lens has a radius of curvature of about –6.50 mm. Although the posterior lens surface has a negative radius of curvature, it is still a convex surface which adds optical power to the eye, and relatively more so than does the anterior lens surface since the lens posterior surface is more steeply curved than the lens anterior surface. The lens anterior and posterior surface curvatures (as well as the lens gradient refractive index) are important to the optical power of the eye and it is these surfaces that become more steeply curved to allow the accommodative increase in optical power of the lens to occur. Historically it was suggested that the posterior lens surface does not move and that the posterior lens surface curvature does not change appreciably with accommodation. However, it is now known that the posterior lens surface does undergo an increase in curvature and moves posteriorly during accommodation as the lens thickness increases. Gullstrand suggested that the lens equivalent refractive index must change during accommodation. Since the lens shape, axial thickness and equatorial diameter change during accommodation, this dictates that the form of the gradient refractive index of the lens must also change during accommodation. Although the form of the lens gradient refractive index changes as the lens changes shape during accommodation, this does not require a change in the equivalent refractive index of the lens during accommodation, at least to the extent that resolution limits of currently available technology permit this to be discerned.
The optical requirements for accommodation
The optical power of the crystalline lens increases (i.e. the lens focal length decreases) during accommodation. As a consequence, the eye changes focus from distance to near so the image of a near object is brought to focus on the retina. The dioptric change in power of the eye defines accommodation and accommodation is measured in units of diopters (D). A diopter is a reciprocal meter and is a measure of the vergence of light. Light rays from a point object diverge and are by convention designated to have negative vergence. Light rays converging towards a point image are designated to have positive vergence (see Chapter 1 ). An object at optical infinity subtends zero vergence at the cornea. The optical interfaces of the eye (the cornea and lens) add positive vergence to draw light rays towards a focus on the retina ( Fig. 3.3 ). When an object is moved from infinity to a point closer to the eye, the near object subtends divergent rays on the cornea. To focus on the near object, the optical power of the eye must increase to add positive vergence to the now divergent rays to bring the refracted rays to a focus on the retina. When an emmetropic eye is focused on a distant object the eye is considered unaccommodated. If the eye accommodates from an object at optical infinity to an object 1.0 m in front of the eye, this represents 1.0 D of accommodation. If the eye accommodates from infinity to 0.5 m in front of the eye, this is 2 D of accommodation; from infinity to 0.1 m is 10 D, and so on. The accommodative response is therefore the increase in optical power that the eye undergoes to change focus from an object at optical infinity to the near object.

Depth of field
Clinically, the nearest point of clear vision is typically measured subjectively in an eye corrected for distance vision. This is done by moving a near reading chart towards the eyes while the subject is asked to report when they can no longer sustain clear vision on the near target or when the near target first becomes blurred. Although the reciprocal of this near reading distance expressed in meters is clinically referred to as the accommodative amplitude, this is technically inaccurate. The push-up test is a subjective measure of the difference between the far point and the near point expressed in units of diopters. However, this is not a measure of the true dioptric change in power of the eye because of the depth of field of the eye. Depth of field is defined as the range over which an object can be moved towards or away from the eye in object space without a perceptible change in the blur or focus of the image. The depth of field of an eye depends on many factors. For example, depth of field is dependent on pupil size. A large pupil results in a wide and steep cone of light converging towards the retina. A small error in image focus with respect to the position of the retina therefore results in a large change in image blur. A large pupil results in a relatively small depth of field. A small pupil results in a narrow and flat cone of light converging towards the retina. Small errors in image focus with respect to the position of the retina result in relatively small changes in image focus. A small pupil therefore results in a relatively larger depth of field.
The depth of field of an eye is dependent on the level of illumination because of the effect that illumination has on pupil diameter. For a brightly illuminated object, pupil size will decrease resulting in an increase in depth of field. The presence of optical aberrations such as astigmatism, coma and spherical aberration also act to increase the depth of field of an eye. The presence of ocular aberrations results in an image that is not in sharp focus on the retina. Therefore, small movements of the object in object space would not perceptibly alter the focus of the image on the retina in an eye with aberrations. With accommodation and with increasing age the pupil size decreases. An effort to focus at near therefore increases the depth of field of the eye due to pupil constriction. When the nearest point of clear vision is assessed using subjective methods, such as the push-up or push-down method, the depth of field of the eye results in an overestimation of the dioptric change in optical power of the eye. When the near point of clear vision is measured using a subjective push-up method, this overestimates the objectively measured accommodative response amplitude by about 1–2 D. Subjective testing of this nature in complete presbyopes might lead one to believe that about 1 D of accommodation is present, but this is not a true change in optical power of the eye and is therefore called pseudoaccommodation.
Visual acuity
In addition to the depth of field of the eye, acuity or contrast sensitivity of the eye also affect the subjective measurement of the near point of clear vision. The subjective push-up measurement relies heavily on the subject perceiving when the object can no longer be seen in sharp focus. As a near reading target is brought closer to the eye, the subject must decide at what point an object is no longer in acceptable focus. As mentioned, the level of illumination of the target can affect the depth of field of the eye, but illumination also affects the contrast and the brightness of the image. If the target is viewed in dim illumination, it is more difficult to detect when it is in clear and sharp focus. A brightly illuminated reading target will be seen more clearly. The increased illumination provides higher contrast on the target and so smaller changes in focus or blur of the target are more easily detected. While increasing the level of illumination will help to improve the contrast sensitivity and acuity, this will also decrease the pupil size and will thereby increase the depth of field of the eye and so result in a nearer point of perceived clear vision. Further, in cases of cataract or other opacities of the ocular optical media, the image of a near object is not seen clearly and so small changes in the focus of the image are less readily detected. With increasing age the optical clarity of the lens decreases and the prevalence of cataract increases. Retinal disease can also affect visual acuity. Elderly patients often have reduced visual acuity and/or reduced contrast sensitivity, although not solely due to decreased optical performance. If the near point of clear vision is measured using the subjective push-up test in presbyopes or in patients with cataracts or retinal disease, this will overestimate the true objectively measured accommodative amplitude.
The anatomy of the accommodative apparatus
The accommodative apparatus of the eye consists of the ciliary body, the ciliary muscle, the choroid, the anterior and posterior zonular fibers, the lens capsule and the crystalline lens ( Fig. 3.4 ). Theoretical suggestions for a role for the vitreous in accommodation and empirical evidence against a need for the vitreous in accommodation exist. The ciliary muscle is located within the ciliary body beneath the anterior sclera. The ciliary muscle is comprised of three muscle fiber groups oriented longitudinally, radially (obliquely) and circularly. The anterior zonular fibers span the circumlental space extending from the ciliary processes to insert all around the lens equator. These zonular fibers constitute the suspensory elements of the crystalline lens. Posterior zonular fibers extend between the tips of the ciliary processes and the pars plana of the posterior ciliary body near the ora serata. The crystalline lens consists of a central nucleus and a surrounding cortex. This lens is surrounded by the collagenous elastic lens capsule.

The ciliary body
The ciliary body is a triangular-shaped region bounded on its outer surface by the anterior sclera and on its inner surface by the pigmented epithelium. It lies between the scleral spur anteriorly and the retina posteriorly. The anterior ciliary body begins at the scleral spur at the angle of the anterior chamber. The base of the iris inserts into the anterior ciliary body. Posterior to the iris, the ciliary processes are found at the anterior-innermost point of the ciliary body and form the corrugated pars plicata of the ciliary body. Posterior to the pars plicata the smooth surface of the ciliary body is called the pars plana. The vitreal surface of the pars plana is spanned by longitudinally oriented posterior zonular fibers. The most posterior aspect of the ciliary body joins to the ora serrata of the retina. The outer surface of the ciliary body beneath the anterior sclera is the suprachoroidal lamina or supraciliarus, formed by a thin layer of collagen fibers, fibroblasts and melanocytes. Ultrastructural differences exist between the ciliary non-pigmented epithelial cells at the tips of the processes and those in the valleys, the former being adapted for fluid secretion and the latter for mechanical anchoring of the zonule. The length of the ciliary body from the tips of the ciliary processes to the ora serrata is longest temporally and shortest nasally.
The ciliary muscle
The ciliary muscle occupies a triangular-shaped region within the ciliary body beneath the anterior sclera ( Fig. 3.5 ). It has an anterior origin at the scleral spur in close proximity to Schlemm’s canal. Anterior ciliary muscle tendons insert into the scleral spur and the trabecular meshwork, which serve as a fixed anterior anchor against which the ciliary muscle contracts. Posterior to the scleral spur, the outer surface of the ciliary muscle is attached only loosely to the inner surface of the anterior sclera. The posterior attachment of the ciliary muscle is to the stroma of the choroid. The anterior and inner surfaces of the ciliary muscle are bounded anteriorly by the stroma of the pars plicata and posteriorly by the pars plana of the ciliary body. The ciliary muscle fiber bundles beneath the sclera are oriented such that a contraction of the ciliary muscle results in a forward and inward redistribution of the mass of the ciliary body and a narrowing of the ciliary ring diameter due to sliding ciliary muscle movement along the inner surface of the sphere formed by the anterior sclera. This causes the anterior choroid to be pulled forward. The ciliary muscle is a smooth muscle, with a dominant parasympathetic innervation causing contraction mediated by M3 muscarinic receptors and a sympathetic innervation causing relaxation mediated by β 2 -adrenergic receptors. The ciliary muscle is atypical for smooth muscles, in its speed of contraction, the large size of its motor neurons, the distance between the muscle and the motor neurons, and the unusual ultrastructure of the ciliary muscle cells which in some ways resemble skeletal muscles (indeed, in birds it is a striated skeletal muscle).

There are also regional differences in ultrastructure and histochemistry of the primate ciliary muscle, suggesting that the longitudinal portion may be acting like a fast skeletal muscle to “set” or “brace” the system rapidly, for the contraction of the inner portion to be most effective. The ciliary muscle is comprised of three muscle fiber groups identified by their relative positions and orientations, forming a morphologically and functionally integrated three-dimensional structure. The major group of muscle fibers is the peripheral meridional or longitudinal fibers or Brücke’s muscle. They extend longitudinally between the scleral spur and the choroid adjacent to the sclera. Located inward to the longitudinal fibers are the reticular or radial fibers. These constitute a relatively smaller proportion of the ciliary muscle. The radial fibers are branching V- or Y-shaped fibers. These radial fibers are attached anteriorly to the scleral spur and the peripheral wall of the anterior ciliary body at the insertion of the iris. They attach posteriorly to the elastic tendons of the choroid. Beneath the radial fibers and positioned more anteriorly in the ciliary body and closest to the lens are the equatorial or circular fibers or Müller’s muscle. These constitute the smallest proportion of the ciliary muscle.
The division of the ciliary muscle into three muscle fiber groups is somewhat artificial. In reality, there is a gradual transition from the outermost longitudinal muscle fibers to the radial fibers to the innermost circular muscle fibers with some intermingling of the different fiber types. A contraction of the ciliary muscle results in a contraction of all three muscle fiber groups together. With a contraction of the ciliary muscle there is a gradual rearrangement of the muscle bundles, with an increase in thickness of the circular portion and a decrease in thickness of the radial and longitudinal portions. A contraction of the entire ciliary muscle as a whole pulls the anterior choroid forward, moves that apex of the ciliary processes towards the lens equator and serves the primary function of releasing resting zonular tension at the lens equator to allow accommodation to occur.
The zonular fibers
The zonular fibers are a complex meshwork of fibrils. Fibrils 70–80 nm in diameter are grouped into fiber bundles estimated to be between 4–6 to 40–50 micrometers in diameter. The zonule is composed of the non-collagenous carbohydrate-protein mucopolysaccharide and glycoprotein complexes that are secreted by the ciliary epithelium. The zonular fibers are elastin-based elastic fibers and are thought to be much more elastic than the lens capsule. Their primary function is to stabilize the lens and allow accommodation to occur. Since the zonule is not a continuous tissue, but is composed of fibers, it also allows fluid flow from the posterior chamber behind the iris through to the vitreous chamber (see Chapter 11 ).
The attachment of the zonular fibers to the lens capsule is superficial with few fibers penetrating into the capsule to form a mechanical (possibly similar to Velcro) or chemical union. From scanning electron microscopy this anterior zonule crossing the circumlental space and extending to the lens is alternatively described as:
- (i)
consisting of three fiber strands running to the anterior, equatorial and posterior lens surfaces, or
- (ii)
fibers that insert along a circular line on the anterior and posterior surface of the lens with some fibers inserting directly on the equator, or
- (iii)
a zonular fork with two main fiber groups extending to the lens anterior and posterior surfaces with finer bundles seemingly of relative unimportance running to the lens equator, or
- (iv)
successive sagittal lines of insertion from lens anterior to posterior surface and two coronal lines of insertion, one where the fibers insert onto the capsule around the anterior surface and another where the fibers insert onto the capsule around the posterior surface.
Although no systematic crossing of anterior zonular fiber was observed by McCulloch, crossing of anterior zonular fibers has been observed in other preparations and was documented in early diagrams from histology of this tissue ( Fig. 3.6 ). From histological preparations, when an appropriate plane of section is obtained, a continuous line of zonular insertion into the entire lens equator is seen. Unfixed, dissected human eye specimens show a continuous meshwork of fibers uniformly covering the entire lens equator, and show crossing of zonular fibers.

Observations of the ciliary region during accommodation show that the posterior ciliary body slides forward against the curvature of the anterior sclera, moving the posterior insertion of the posterior zonular fibers forward. However, contraction of the ciliary muscle stretches the posterior attachment of the ciliary muscle due to a forward and inward movement of the tips of the ciliary muscle and ciliary processes. This suggests that the posterior zonular fibers may similarly assist in pulling the ciliary muscle back to the unaccommodated configuration after cessation of an accommodative effort.
The lens capsule
The crystalline lens is surrounded by the lens capsule ( Fig. 3.6 ). This is a thin, transparent, elastic membrane secreted by the lens epithelial cells largely composed of collagen type IV. Fincham was the first to attribute the accommodative change in shape of the lens to the forces exerted on the young lens by the lens capsule. Fincham studied the capsule in histological section and found it to be of relatively uniform thickness in non-accommodating mammals. However, in primates Fincham found it to be thickest at the mid-peripheral anterior surface, thinner towards the lens equatorial region with a posterior peripheral thickening, but thinnest at the region of the posterior pole of the lens ( Fig. 3.7 ). Several aspects of Fincham’s idealized description of the capsule have been largely confirmed in a more recent study, although with some age-related changes in thickness. The capsule is about 11–15 µm thick at the anterior pole. There is an anterior, mid-peripheral thickening of the capsule that is about 13.5–16 µm thick. This is located more central to the region of zonular insertion into the capsule around the lens equator. The equatorial region of the capsule, to which the anterior zonular fibers insert, is about 7 µm thick at the lens equator and does not appear to change systematically with age. The posterior capsule thickness decreases to a minimum at the posterior pole of about 4 µm, without a posterior mid-peripheral thickening ( Fig. 3.8 ).


The crystalline lens
The lens consists largely of lens fiber cells composing the nucleus and cortex. On the anterior lens surface beneath the capsule is a layer of lens epithelial cells. The embryonic nucleus remains present at the center of the lens throughout life as the cortex grows progressively around it by the addition of an increasing number of layers of lens fiber cells. The deeper layers of lens epithelial cells on the lens anterior surface differentiate to become lens fiber cells. The proliferation of lens epithelial cells and their differentiation into lens fiber cells continues throughout life. Because the lens is contained within the capsule, lens epithelial cells do not slough off as do epithelial cells in other organ systems such as those lining the skin and gut. Therefore, the lens continues to grow throughout life. After adolescence the human lens undergoes a linear increase in mass with increasing age. In vivo, with increasing age, lens thickness increases with a resulting increase in the anterior surface and posterior surface curvatures. Although lens thickness and surface curvatures change systematically with increasing age, this occurs without a systematic age-related change in lens diameter.
The crystalline lens has a gradient refractive index, with a refractive index of 1.385 near the poles and a higher refractive index of 1.406 at the center of the nucleus. The lens is not optically homogeneous and when viewed through a slit-lamp, several optical zones of discontinuity are observed which allow visual differentiation of the lens nucleus from the surrounding lens cortex ( Fig. 3.9 ). The unaccommodated young adult human lens is roughly 9.0 mm in diameter and 3.6 mm thick. The lens thickness increases by approximately 0.5 mm with 8 D of accommodation.

The mechanism of accommodation
Current understanding of the accommodative mechanism is largely in accord with the description provided by Helmholtz in 1855 ( Fig. 3.10 ). Although Fincham and more recent investigations have added further to understanding the accommodative mechanism, the basic tenets are in accord with those originally described by Helmholtz. When the young eye is unaccommodated and focused for distance, the ciliary muscle is relaxed. Resting tension on the zonular fibers spanning the circumlental space and inserting around the lens equator (collectively called the anterior zonular fibers ) apply an outward directed tension around the lens equator through the lens capsule to hold the lens in a relatively flattened and unaccommodated state. For the eye to focus at near, the ciliary muscle contracts, the inner apex of the ciliary body moves forward and towards the axis of the eye ( Fig. 3.11 ). This inward movement of the apex of the ciliary muscle stretches the posterior attachment of the ciliary muscle and releases resting tension on all zonular fibers around the lens equator. The lens capsule then molds the lens into a more accommodated form. The capsule provides the force to cause the lens to become accommodated. A clear role for the lens capsule in accommodation stems from observations by Graves of the effect of accommodation on the empty lens capsule in an otherwise healthy aphakic eye in which the lens was absent. When the patient looked at a distant object, the anterior and posterior capsule was taught and flat. When the patient focused on a near object, the capsule became mildly flaccid and the surfaces separated. An eserine stimulated contraction of the ciliary muscle resulted in a completely slack capsule (see Fig 3.7 ). Fincham concluded that resting zonular tension at the lens equator pulls outward on the capsule to hold the lens in the unaccommodated state and when the eye is accommodated, the resting tension on the zonular fibers is released to allow the capsule to mold the lens into the accommodated form.


Further evidence of the role of the capsule comes from in vitro experiments. In dissecting a young human or monkey eye, when the zonular fibers around the lens equator are cut, the isolated young lens with the intact capsule assumes a maximally accommodated form. If the capsule is then cut and carefully removed from the isolated lens, the young decapsulated lens substance assumes a maximally unaccommodated form. Further, mechanical stretching studies of partially dissected young human and monkey eyes show that applying an outward directed stretching force to the lens via the capsule and anterior zonular fibers will pull the lens into a flattened and unaccommodated state and releasing the zonular tension will allow the lens to become accommodated through the forces exerted by the capsule on the lens ( Fig. 3.12 ). Such in vitro mechanical stretching studies reliably and reproducibly produce accommodative optical changes in the lens that match the accommodative optical changes in vivo in the living eye.

During accommodation, lens diameter decreases systematically in response to voluntary accommodation, brain stimulated accommodation or pharmacologically stimulated accommodation ( Figs 3.13, 3.14 & 3.15 ). Lens thickness increases and the central anterior surface curvature and to a lesser extent the central posterior surface curvature increases. These physical changes in the lens are relatively linearly correlated with the accommodative optical changes in the eye ( Fig. 3.16 ). The increased lens surface curvature results in an increase in the optical power of the crystalline lens. Anterior chamber depth decreases due to the forward movement of the anterior lens surface and the vitreous chamber depth decreases due to the posterior movement of the posterior lens surface ( Fig. 3.16 ). About 75 percent of the increase in lens thickness is accounted for by the anterior movement of the anterior lens surface and about 25 percent of the increase in lens thickness is accounted for by a posterior movement of the posterior lens surface.




When the accommodative effort ceases, the ciliary muscle relaxes and the elasticity of the posterior attachment of the choroid pulls the ciliary muscle back into its flattened and unaccommodated configuration. The outward movement of the apex of the ciliary body once again increases the tension on the anterior zonular fibers around the lens equator to pull the lens via the capsule into a flattened and unaccommodated form.
Variants on the Helmholtz accommodative mechanism have been suggested to include an essential role for the vitreous and differential pressure changes in the eye. However, accommodation still occurs after vitrectomy and mechanical stretching studies of dissected eyes in which the vitreous is absent and no pressure differential can exist, results in normal accommodative optical changes to the lens, thereby obviating a role for the vitreous or for differential pressure changes in the eye. A revisionist theory of accommodation originally proposed by Tscherning has been espoused. This theory is opposite to the Helmholtz accommodative mechanism in that it paradoxically requires an increase in lens equatorial diameter during accommodation, a flattening of the peripheral lens surfaces and an increase in curvature of the lens central surfaces. However, there exists no experimental evidence in support of the supposed accommodative increase in lens diameter. In fact, numerous studies demonstrate that lens diameter decreases systematically during accommodation.
Accommodative optical changes in the lens and eye
For the unaccommodated emmetropic eye to focus on a near object requires an increase in optical power of the eye. This occurs through an increase in optical power of the lens. The accommodative increase in optical power of the lens comes about from an increase in the lens anterior and, to a lesser extent, posterior surface curvatures. Several other physical changes in the eye and lens also occur during accommodation that have optical effects on the eye. Lens thickness increases, the lens anterior surface moves anteriorly to reduce anterior chamber depth and the lens posterior surface moves posteriorly to increase anterior segment length. The lens asphericity changes and the pupil constricts. In addition, because the lens has a gradient refractive index, the form of which is constrained by the shape and size of the lens, since the lens changes shape, so too must the form of the lens gradient refractive index. All of these accommodative physical changes in the eye and lens result not only in an increase in optical power, but also in other changes in the ocular aberrations of the eye.
Simple paraxial vergence calculations show that for parallel rays incident on a simple biconvex lens, if lens thickness alone is increased, lens power decreases. In an eye, however, if the lens thickness increases, this must occur in conjunction with either a decrease in anterior chamber depth or an increase in anterior segment length consequent to the increase in lens thickness. For a distant object, the lens inside the eye does not have parallel light incident on it, but rather convergent light due to refraction by the cornea. Simple paraxial schematic eye calculations show that if lens thickness alone is increased without a change in lens curvatures but with a resultant decrease in anterior chamber depth, the result is an overall increase in power of the eye. The accommodative increase in optical power of the lens however is primarily due to an increase in the lens surface curvatures.
Since the lens anterior surface is flatter than the lens posterior surface, for a given change in curvature the anterior surface will undergo a relatively greater increase in optical power than the posterior surface. The accommodative optical increase in power of the eye is therefore ultimately due to a complex combination of optical and physical changes in the lens and the eye. This results not only in an increase in optical power of the eye, but also accommodative changes in ocular aberrations of the eye. In particular, accommodation is accompanied by an increase in negative spherical aberration of the eye and lens ( Figs 3.17 & 3.18 ). In addition, the iris constricts during accommodation, to decrease the optical entrance pupil of the eye. This too has optical effects. Simply decreasing the entrance pupil diameter results in an overall reduction in optical aberrations of the eye. Further, in an eye with negative spherical aberration, in which the paraxial rays are focused closer to the lens than the peripheral rays, a pupil constriction alone would result in an overall increase in optical power of the eye simply due to the constricted iris occluding the more weakly refracted peripheral rays. In a young eye, when accommodation occurs, all these various optical changes occur in concert.
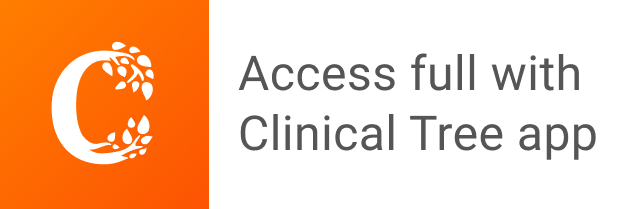