5 Optical Coherence Tomography
5.1 Introduction
Optical coherence tomography (OCT) is a noninvasive diagnostic imaging technology that renders in vivo cross-sectional images of light-scattering media with micron resolution. OCT is based on the principles of optical interferometry, which is a technique of extracting information about waves based on constructive or destructive inference patterns when two waves are superimposed.
5.2 Brief History
OCT was first reported in 1991 through a collaboration between clinicians, engineers, researchers, and students. 1 After further development of the software and hardware, in vivo retinal imaging was reported in 1993 at Tufts Medical Center, New England Eye Center, Boston, MA. 2 , 3 , 4 Carl Zeiss Meditec introduced the first commercial OCT device in 1996 and a second-generation device in 2000, with successive advances in both hardware and software capabilities. It was in 2002 with the release of the Stratus OCT (Carl Zeiss Meditec) that the technology became widely accepted in the ophthalmologic community. In 2006, the first spectral-domain OCT (SD-OCT) device was released by Optovue, Inc., allowing for higher resolution scans and faster scanning speed compared to the Stratus OCT. Over the next few years, several other manufacturers released various SD-OCT devices with differing scanning parameters, speeds, and software capabilities.
Over time, OCT has become integral in elucidating in vivo microscopic changes in ocular structures, particularly with retinal pathology. The influence of the technology is demonstrated by the number of reports and publications on OCT, with approximately 3,500 publications in 2013 alone and more than 17,000 publications from 2000 until the present time.
5.3 Principles
The initial OCT prototype was based on the Michelson interferometer in which a source emits low-coherence light into a beam splitter, with one arm directed to a reference mirror and the other arm to the sample of interest, or tissue. The resulting backscattered lights from the tissue and reference mirror are combined and analyzed for interference patterns of intensity, providing measurements of the depth and reflectivity of tissue structures at a given point (A-scan). Low-coherence light is utilized to decrease the distance over which interferometric signals are able to be detected, from meters to micrometers. However, the use of low-coherence light also requires the backscattered lights from the reference mirror and sample to be of comparable length, within one wavelength, in order to detect interferometric signals. Multiple A-scans are combined along a linear path to create a cross-sectional slice of the tissue (B-scan, or “line scan”), and likewise multiple B-scans can be combined to provide three-dimensional images (C-scan).
Three methods for the detection of interferometric signal have been developed into commercial systems.
5.3.1 Time-Domain OCT
Time-domain (TD) systems acquire interferometric signals by mechanically moving the reference mirror to correspond to the length of backscattered light in the tissue sample for each individual A-scan. TD-OCT systems were able to achieve rates of 400 A-scans per second with axial resolutions of 8 to 10 µm. 5
5.3.2 Spectral-Domain OCT
SD technology, a type of Fourier-domain OCT, utilizes a fixed reference arm and a broadband light source to produce a spectrum of wavelengths of backscattered light, which is measured by a high-speed spectrometer in the detection arm of the interferometer. 6 This information is converted by inverse Fourier transformation to provide multiple A-scans with a single exposure. The collection of data simultaneously from each location improved sensitivity and sampling speed, generating 18,000 to 70,000 A-scans per second with axial resolution of 5 to 7 µm, several orders of magnitude faster and more sensitive than TD-OCT. 7 , 8
5.3.3 Swept-Source OCT
Also referred to as time-encoded frequency-domain OCT, this technology utilizes a tunable frequency swept laser light source instead of a reference mirror to sweep through a broad range of frequencies. The interferometric signals are detected on a single or small number of receivers as a function of time and undergo inverse Fourier transformation to generate A-scan information. Speeds of up to 400,000 A-scans per second have been achieved with swept-source OCT (SS-OCT).
5.3.4 Comparison of OCT types
TD-OCT
The Stratus OCT (Carl Zeiss Meditec) is the primary commercially available scanner, which was released in 2002. It is the device with the longest history of use and has been utilized in the largest number of published studies of all OCTs available. However, being a TD-OCT system, it scans at a slower rate, resulting in more motion artifacts and covering a smaller portion of the retina. Additionally, the lower resolution of TD-OCT compared to SD-OCT and SS-OCT does not permit visualization of finer retinal structures or pathology, particularly the outer photoreceptor layers, retinal pigment epithelium (RPE), or choroid. It also suffers from lower interscan repeatability compared to SD-OCT. 9
The main retinal scan output for this device consists of six radial line scans, centered on the fovea, separated 30 degrees apart. Owing to the amount of spacing between each line scan, retinal pathology falling in between line scans can easily be missed. The system outputs retinal thickness measurements, which have been reported in numerous studies. However, thickness measurements on the Stratus cannot be directly compared to SD-OCT scanners since the segmentation of the outer retina is different between the two types of scanners. 9
SD-OCT
There are many commercially available SD-OCT scanners, with various scanning patterns, scanning speed, resolution, and additional modes (e.g., anterior segment OCT). The higher speed of SD-OCT scans decreases motion and blink artifacts seen with TD-OCT. Additionally, the higher scan speeds allow for denser sampling of the retinal area, decreasing the chance of missing retinal pathology. The improved resolution allows for better visualization of retinal structures such as the outer photoreceptor bands from the RPE as well as subtle retinal pathology.
Hand-held, portable SD-OCT devices have been developed, which are useful for intraoperative scanning or for patients who have positioning difficulties. 10 , 11 An intraoperative OCT device that attaches to the operating microscope has been developed. 12
SS-OCT
SS-OCT provides higher scanning speeds (typically between 100,000 and 250,000 A-scans per second) than SD-OCT and has less loss of sensitivity with depth changes, allowing for improved visualization of both deep structures (i.e., choroid) and the vitreoretinal interface. 13 , 14 Additionally, the faster scan speeds and increased depth range allow for a larger peripheral range than SD-OCT and TD-OCT.
5.4 Types of Scans
Multiple analyses and outputs are available across the various systems and software packages, with several general scan patterns available across all commercially available devices. Variables such as B-scan density, speed, length of scan, and pixel density are programmable depending on the machine used. In current practice, there are two main types of scans, macular scans (cube, raster, star patterns) and line scans, as well as other additional scans (detailed later), for analyzing various layers of the retina in specific diseases.
5.4.1 Cube Scan
Macular cube scans are compilations of a series of B-scans to create a volumetric cube of data, similar to computed tomography scans. The scans generally cover a 6 × 6 mm area centered on the fovea and are of lower resolution than line scans in order to decrease the total scan time required. The center of the scan can be manually moved to a different area of the retina by the operator and can be output as a topographic map with a color-coded Early Treatment Diabetic Retinopathy Study (ETDRS) grid (Fig. 5-1). Various scanners use different protocols, with some offering lower density B- and C-scans as “fast” options. A more significant difference that plays a role in analysis is the type of grid scan used.

5.4.2 Raster Scan
Raster scans involve a rectangular grid consisting of closely spaced parallel B-scans of the area of interest. This type of scan acquires a uniform amount of information of the area scanned, with an equal density of B-scans in the fovea and the outer macula.
5.4.3 Grid Scan
The MM5 protocol of the RTVue device is a mix of vertical and horizontal B-scans to create a grid, rather than a series of parallel B-scans. Specifically, the scan is composed of a less dense 11 × 11 B-scan grid over a 5 × 5 mm area, with each line composed of 668 A-scans, and a denser inner grid of 6 × 6 B-scans covering a 3 × 3 mm area, with each line composed of 400 A-scans. This pattern requires the least amount of interpolation between individual line scans, thereby minimizing the chances of missing pathology.
5.4.4 Radial Scan
Radial scans consist of either 6 or 12 radial high-resolution 6-mm line scans, all of which pass through the fovea. This was the primary scanning pattern of the Stratus TD-OCT and is offered on the RTVue, Topcon 3D, and Heidelberg Spectralis devices. The advantage compared to raster scanning is that with a given number of B-scans, the individual line scans are more closely spaced in the foveal area where visually significant pathology is likely located. This is similar to grid scanning, but has a speed advantage since additional scans do not need to be taken to increase the density of line scans in the foveal area. However, the disadvantage is that the software interpolates between each radial scan and may miss pathology located in the outer macular area where the radial line scans are farther apart.
5.4.5 Line, High-Definition Line, and Cross-Line Scans
Line scans (Fig. 5-2) are individual B-scans composed of a higher density of A-scans compared to cube scans, resulting in higher resolution. This is particularly useful for delineating specific retinal layers and identifying subtle pathology in a known area of interest. The Zeiss Cirrus features a 6-mm five-line raster, with each line sampled four times and averaged. Additionally, the five lines can be collapsed into one line with an average of 20 samples to create a “high-definition” OCT line scan by image averaging. Image averaging averages multiple B-scans of the same retinal location to increase the signal-to-noise ratio. The RTVue system additionally features a cross-line scan, consisting of horizontal and vertical line scans, like a higher definition mesh scan. The Heidelberg Spectralis features a seven-line raster scan.

5.4.6 Enhanced Depth Imaging
Enhanced depth imaging (EDI) was first reported by Spaide et al in 2008 and is now available in all commercial OCT devices. 15 EDI better visualizes the scleral–choroidal border (Fig. 5-3, arrows), allowing for measurements of choroidal thickness and analysis of lesions affecting the choroid, which would not be visible on standard line scans due to the attenuating effects of the RPE. EDI is a method of scanning in which the objective of the lens of the OCT is placed closer to the eye of the patient, resulting in an inverted image and centering the zero-delay line closer to the choroid rather than the retina.

5.4.7 Layer Specific
Various devices feature the ability to analyze specific layers of the retina using algorithms to determine the inner and outer borders of those layers. For accurate identification of the borders of the retinal layer of interest, a high-quality scan with high signal strength is required. Two analyses in particular have been reported and are used to follow specific diseases: retinal nerve fiber layer (RNFL) analysis and ganglion cell layer (GCL) analysis.
Retinal Nerve Fiber Layer
The retinal nerve fiber layer (RNFL) analysis measures the RNFL circumferentially at a radius of 1.7 mm centered on the optic nerve. It is used primarily to follow glaucoma progression, but is also used in diseases of the optic nerve such as optic neuritis, optic atrophy, nonarteritic optic neuropathy, and papilledema. 16 , 17 , 18 , 19 , 20 The output is displayed as segmented measurements of nerve fiber layer thickness as well as a color-coded graph with a comparison to age-matched controls (Fig. 5-4).

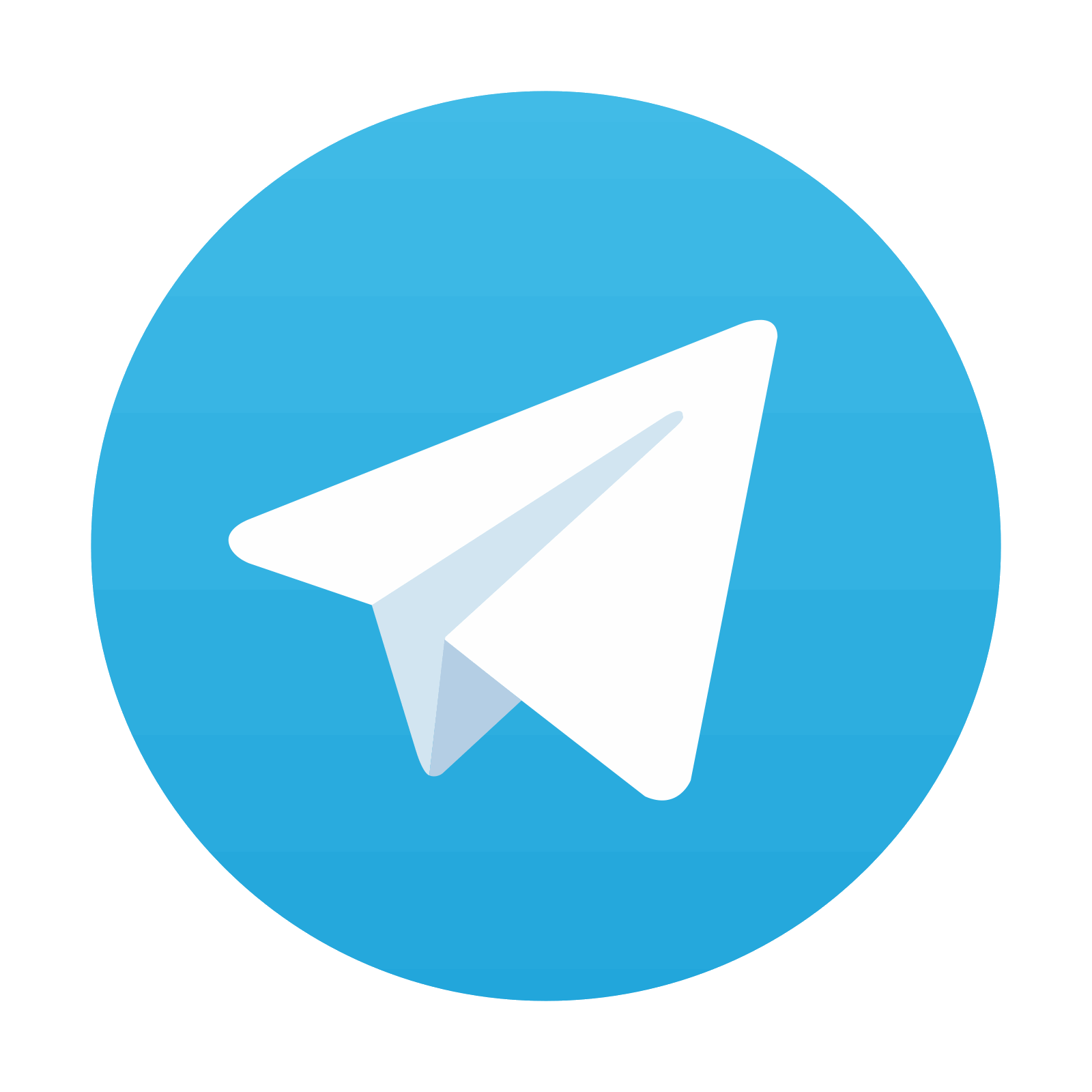
Stay updated, free articles. Join our Telegram channel
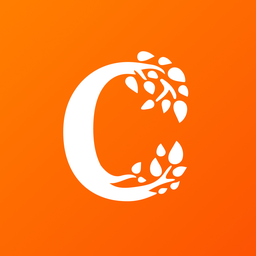
Full access? Get Clinical Tree
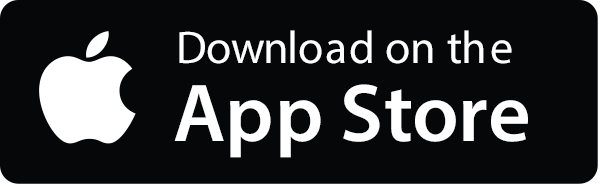
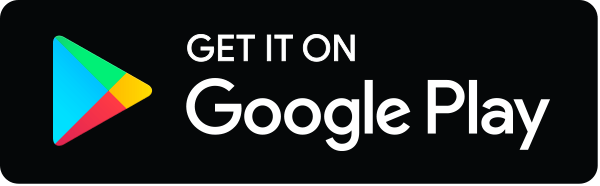
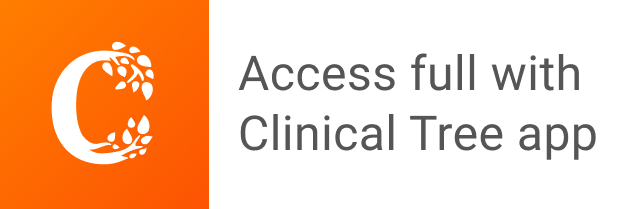