39 Laser for Vitreoretinal Diseases
Retinal laser therapy was developed more than 50 years ago and has had a profound and lasting impact on the treatment of numerous vitreoretinal and choroidal disorders. Since the introduction of the ruby laser in 1960, 1 the safety and efficacy of retinal lasers have been investigated in several large clinical trials. Significant advances have been achieved in laser technology and the molecular understanding of laser–tissue interactions to minimize collateral tissue damage while retaining the therapeutic effects of laser therapy. This chapter gives a brief history of retinal lasers, discusses the science behind lasers and laser–tissue interactions, reviews delivery systems and current clinical applications and trials evaluating lasers, and discusses promising recent innovations in retinal laser therapy. The important clinical role of lasers is highlighted in proliferative diabetic retinopathy (PDR), diabetic macular edema (DME), central retinal vein occlusions (CRVOs), branch retinal vein occlusions (BRVOs), central serous chorioretinopathy (CSCR), choroidal neovascularization (CNV), retinal tumors, polypoidal choroidal vasculopathy, retinal tears, and numerous additional retinal conditions.
39.1 History
In 1900, Max Planck published the relationship between energy and the frequency of radiation. In 1917, Albert Einstein proposed the concept of stimulated emission; that is, electrons could be stimulated to emit light of a particular wavelength, creating the theoretical framework for the development of the laser. 2 The first device to utilize these concepts was not developed until 1954, when American physicists Charles H. Townes, Herbert J. Zeiger, and James P. Gordon produced the first MASER (microwave amplification by stimulated emission of radiation) at Columbia University. In March 1960, Townes and Arthur L. Schawlow at Bell Labs were granted a patent for a similar device using optical light: a LASER (light amplification by stimulated emission of radiation). In May 1960, Theodore H. Maiman, a physicist at Hughes Research Laboratories in California, constructed the first laser using synthetic ruby and photographic flashlamps as a pump source and, thus, the laser was born. By 1961, several companies were producing commercial lasers. 3
Although lasers were not developed until 1960, 1 retinal photocoagulation dates back to Socrates, who observed solar retinitis or solar eclipse burns of the retina. Czerny, in 1867, 4 and Deutschmann, in 1882, 5 demonstrated burns in rabbit retinas by focusing sunlight through dilated pupils. However, utilizing the sun proved to be suboptimal for medical applications due to its motion, dependence on weather, and large angular size (0.52 degrees). In 1944, Eccles and Flynn 6 provided some insight into the relationship between the time of exposure and the heat intensity required to produce a solar burn in rabbit retinas. In 1955, Byrnes et al 7 demonstrated that rabbit retinas exposed to nuclear explosions had burns similar to those in retinas exposed to sunlight. The following year, Guerry et al 8 and Ham et al 9 carried out quantitative measurements of exposure time and intensity of retinal burns produced with a carbon arc lamp.
In 1945, after noting retinal damage associated with gazing at a solar eclipse, Dr. Gerhard Rudolph Edmund Meyer-Schwickerath 10 surmised that focused radiant energy could be used to create an intentional, therapeutic chorioretinal lesion. He demonstrated that sunlight could be used for this purpose. However, the inability to regulate the power led him to the carbon arc and eventually the xenon arc. 11 By 1956, the xenon arc had gained widespread acceptance, proving to be a convenient source of intense, polychromatic white light emitting all wavelengths from 400 to 1600 nm and producing a full-thickness chorioretinal burn without selective tissue targeting.
The xenon arc has since been supplanted by the more selective laser. The laser emits a discrete wavelength and has a high degree of collimation, permitting a focused, smaller spot size. Clinical investigation with Maiman’s ruby laser was carried out in 1963 by Kapany et al 12 and Zweng et al. 13 Difficulty in producing a full-thickness retinal burn and the risk of subretinal hemorrhage with a pulsed laser were attributed to the 2-ms pulse duration and the emitted red wavelength; this stimulated the development of the continuous-wave (CW) gas ion lasers for use in ophthalmology.
The argon blue-green laser was introduced by Dr. Francis A. L’Esperance in 1968, 14 and it rapidly gained wide acceptance by the mid-1970s for its several advances over the ruby laser. This was followed by the krypton laser in 1972 15 and the indirect laser ophthalmoscope in 1981. 16 The tunable organic dye laser was introduced to ophthalmology in 1981, initially as a source of photodynamic therapy (PDT) and then for photocoagulation therapy. The dye laser enabled variation of output wavelength to target specific ocular tissues.
The semiconductor laser diode was developed in 1962, but clinical applications were limited by low power outputs. Advances in technology led to the development of the gallium aluminum arsenide laser diode, which can emit CW, monochromatic, coherent laser light in excess of 1 W. Emission from this laser is in the near-infrared range with wavelengths between 780 and 840 nm. In 1987, Puliafito et al 17 first performed retinal photocoagulation lesions using a diode laser endophotocoagulation delivery system. McHugh et al 18 in 1989 described the use of clinical transpupillary retinal photocoagulation with the diode for treating a variety of retinal vascular diseases. Diode lasers are now available in many delivery modes: transpupillary slit-lamp biomicroscope and indirect ophthalmoscope, transscleral, and endoprobe.
39.2 Laser Principles
LASER (light amplification by stimulated emission of radiation) is achieved by pumping energy into an active medium to stimulate atoms from a lower, more stable state to a higher energy level through the absorption of a discrete quantum of energy. From this excited state, they return to the lower steady state with the emission of energy. With ordinary light sources, the excited atoms emit light independently and in a variety of wavelengths, which is referred to as spontaneous emission. In a laser, stimulated transition releases photons which are in phase and at the same frequency as that of the stimulating light.
Lasers comprise three basic elements: an active medium, a resonant cavity, and an excitation or pumping mechanism. The basic laser cavity is set up by placing an active medium into a resonant cavity with coaxial mirrors at opposite ends (Fig. 39-1). One of the mirrors allows partial transmission of laser light as output. A pumping source introduces energy into the active medium to excite the atoms to a higher energy level and to achieve population inversion. Population inversion refers to the situation in which more atoms reside in a higher energy level than at the lower energy level. Spontaneously emitted radiation occurs in all directions, rarely striking the mirrors. Only stimulated emission strikes the mirror. All stimulated emission is of the same wavelength and in the same direction. In this way, amplified, coherent, collimated light energy is released as laser energy. Laser systems differ in the active laser medium and the way this medium is pumped.

Several qualities of lasers make them a unique source of stimulated emission. These include monochromaticity, spatial coherence, collimation, and ability to produce short pulses. Monochromaticity refers to the ability to emit a very narrow band of wavelengths. Spatial coherence refers to the ability to be focused to a very small spot size. Collimation refers to the lack of divergence of the light waves. These properties allow laser energy to be directed at specific target tissue in a carefully controlled manner.
39.2.1 Laser Output Modes
Lasers can be classified according to the variation of their power output with time. Laser output can be operated in either a continuous wave (CW) or a pulsed mode. A CW laser is one in which the power output undergoes little or no fluctuation with time. There is a steady flow of coherent energy, such as in the helium ion, argon gas, or carbon dioxide lasers. A pulsed mode laser has an output beam power that fluctuates with time, such as an Nd:YAG laser. Pulse duration can range from microseconds (10– 6) to nanoseconds (10– 9), picoseconds (10– 12), or femtoseconds (10– 15). Concentrating energy in short pulse durations results in high peak power. A laser whose output is normally continuous can also be turned on and off to create pulses of light.
There are a variety of ways to produce a short laser pulse. One way is to take a CW laser and mechanically open and close a shutter. It is difficult to produce pulses shorter than 1 µs with mechanical shutters. One disadvantage of this technique is that much of the energy is wasted when the shutter is closed.
A more efficient method to produce short pulses is by pulsing the laser medium itself, for instance, with a flashlamp. Many current solid-state lasers operate in this mode. The shortest flashlamp pulse available is of the order of 1 µs.
Q-switching and mode locking are two methods that allow for compression of the laser output in time to achieve high peak power. The Q-switch is an intracavity shutter that requires an active medium allowing atoms to remain in a high-energy state for a relatively long time to create high peak power. At the appropriate time, the Q-switch shutter is opened, exposing the mirror. Oscillation and stimulated emission follow quickly with the emission of a single, brief, high-power pulse. Q refers to the quality factor of the laser cavity, which is defined as the energy stored in the cavity divided by the energy lost per cycle. Rapid extraction of high power is accomplished as the Q-switch changes the quality factor of the cavity (Q) from high to low. Q-switching can be accomplished electro-optically, acousto-optically, or even mechanically. This is compatible with either a CW or a pulse mode.
Mode locking is a technique whereby ultrashort pulses with picosecond to femtosecond pulse durations can be produced. Normally, a laser oscillates at several frequencies. By synchronizing these modes with a shutter near one of the cavity mirrors, a process called mode locking, even shorter, more powerful pulses can be obtained. Maximum outputs of most ophthalmic models are 10 to 30 mJ and 4.5 mJ for Q-switched and mode-locked lasers, respectively.
39.2.2 Laser Media
Lasers are characterized by their active medium. Depending on the physical state of this substance, lasers are described as solid, liquid, gas, or semiconductor. Moreover, because the spectrum of wavelengths emitted by each element is unique, lasers are named for the excitable medium from which they are generated.
Solid-State Lasers
Solid-state lasers include the ruby, Nd:YAG, and erbium:YAG lasers. The active element in the ruby laser is the chromium ion incorporated into the crystalline sapphire. In the Nd:YAG laser, the yttrium-aluminum-garnet crystal is doped with neodymium ions.
Gas Ion Lasers
Gas ion lasers include the argon, krypton, He-Neon, and carbon dioxide lasers. Ion lasers have an ionized gas as their active medium. Carbon dioxide, in combination with nitrogen and helium, is the active medium in carbon dioxide lasers. Gas discharge lasers do not require a pumping lamp. Instead, a long narrow tube with mirrors at each end contains the gas. The exact wavelength is determined by the coating on the mirrors, which is a good reflector for only one particular wavelength. Disadvantages of gas ion lasers include the need for a special high-voltage power supply and plumbing for water cooling. In addition, they are large, expensive, and not portable.
Liquid Lasers
The operation of tunable dye lasers is based on transitions of organic dye molecules dissolved in solutions. These transitions are broad, and when a series of dye solutions is used, wavelengths from near ultraviolet to near infrared are available. For a given dye, monochromatic output can be obtained by using an intracavity tuning element, usually an etalon, prism, or grating. New solid-state tunable lasers are increasingly popular and are replacing certain tunable dye lasers.
Semiconductor Lasers
Diode lasers are made from semiconductor crystals of gallium arsenide or indium phosphide. The wavelength of the emitted light is a property of the semiconductor crystal. Gallium arsenide doped with aluminum is the most common semiconductor laser crystal in current use and can emit wavelengths between 780 and 850 nm. Other wavelengths can be produced by doping with other materials, but the low power output of these lasers limits their clinical applications. Diode lasers can be used in many delivery modes: transpupillary slit-lamp biomicroscope and laser indirect ophthalmoscope (LIO), transscleral, and endoprobe.
Technical advantages of the diode laser include its compact size and portability. The laser can be powered from an ordinary wall electric current. Additionally, because of their high electric efficiency, little heat is generated and no special plumbing or water cooling system is required. Due to advances in semiconductor technology, diode lasers are relatively inexpensive.
Disadvantages of the diode laser include a nonstandard delivery system with a greater beam divergence cone angle than that of standard argon and krypton lasers. This can make focusing a uniform round laser spot on the retina more difficult. Moreover, infrared laser reflection of up to 10% of the incident beam can occur off the front surface of current laser treatment lenses. This increases the potential danger to unprotected assistants in the treatment room. Standard lenses have antireflective coatings effective in the visible spectrum from 400 to 700 nm. Newer lenses have additional antireflective coatings effective in the near-infrared range.
39.2.3 Laser–Tissue Interactions
The interaction of laser light with a particular target depends on properties of both the laser and the target. The most important laser parameters include wavelength, pulse duration, spot size, and power. The wavelength is a function of the laser cavity’s active medium. According to the principle of wave–particle duality, radiation is propagated in the form of waves and photons. Thus, radiation of a given wavelength is associated with photons of a given energy such that E = hc/?, where h = Planck’s constant, c = speed of light, and ? = wavelength. The frequency and energy increase as the wavelength decreases.
The effects of lasers can be considered in several main categories depending on the parameters of laser wavelength, irradiance, and duration of exposure: photochemical, photothermal, photocoagulation, photodisruption, photovaporization, and photoablation. Photochemical refers to nonthermal light-induced chemical reactions, for example, phototransduction in the retina or photosynthesis in plants. Photothermal refers to light absorption in tissue leading to heating. Photocoagulation, a thermal effect, is the most common therapeutic application for retinal lasers. The main disadvantage of lasers that rely on the mechanism of photocoagulation is their dependence on the absorption of radiation by pigment such as melanin or hemoglobin for absorption of radiation.
Photocoagulation occurs when photon absorption or mechanical vibrations produce a critical temperature rise sufficient to denature biomolecules, resulting in thermally induced structural changes within target tissues. The rise in temperature is proportional to the absorption of light in target tissues. That is determined by the ability of molecules to absorb photons of a given wavelength. The increase in retinal temperature is associated with disruption of hydrogen and van der Waals forces stabilizing molecular configuration. These changes, manifested as a loss of biologic activity or structural integrity, result in cell necrosis, hemostasis, and coagulation. The degree and extent of destruction within a tissue are also related to the laser power, laser absorption, laser spot size, and pulse duration.
Photodisruption and photoablation have joined photocoagulation as established medical laser procedures. 19 Unlike photocoagulation, they do not depend on pigment absorption of radiation. Photodisruption is a process in which high-peak-power, ionizing laser pulses are used to disrupt tissue. Energy is concentrated in time and space to create optical breakdown or ionization of the target medium, with the formation of plasma. Photoablation uses high-energy ultraviolet photons to break molecular bonds directly at the irradiated surface into smaller volatile fragments without heating the remaining substrate, resulting in the removal of tissue.
39.2.4 Tissue Variables
The efficiency of converting light energy to heat energy in a particular tissue depends on how effectively light is absorbed at that site. Several ocular factors determine the effect of the laser on the target tissue. The laser must first be transmitted through the ocular media to reach the target tissue. The ocular media include the cornea, anterior chamber, lens, and vitreous. The cornea may have a stromal scar, the anterior chamber may have a hyphema, the lens may be cataractous, and the vitreous may be hazy secondary to inflammation or the presence of blood. Fluid within or beneath the retina behaves similarly to vitreous haze.
The first law of photochemistry (the Grotthus–Draper law) states that photons must be absorbed by a target for a chemical reaction to be initiated. 20 A chromophore is a molecule that absorbs a photon of a particular energy. Depending on the photon’s energy, a chromophore can undergo bond breakage, ionization, or various types of molecular excitation. In the visible spectrum (400–800 nm), the primary chromophores involved in retinal photocoagulation include: hemoglobin in the red blood cells, macular xanthophyll, melanin in the retinal pigment epithelium (RPE) and pigmented choroid, rhodopsin and cone photopigments in photoreceptors, and lipofuscin in the RPE. The amount and distribution of these pigments in the region of irradiation determine the level of photocoagulation.
Hemoglobin is located in the blood vessels of the inner retina and choroid, as well as in the extravascular tissues. The absorption spectrum of hemoglobin varies with its oxygen saturation. Oxyhemoglobin absorbs maximally at 542 nm (green) and 577 nm (yellow), whereas reduced deoxyhemoglobin absorbs maximally at 555 nm (yellow). Blue, green, and yellow are absorbed well, in contrast to red and infrared, which are absorbed poorly (Fig. 39-2).

Xanthophyll, located within the inner and outer plexiform layers of the macula and in some cataracts, absorbs well below 500 nm. Blue light is absorbed well, green light poorly, and yellow and red light minimally. The use of blue light results in absorption by the inner retina, in addition to the RPE and choroid. Therefore, utilizing blue light for macular photocoagulation can result in damage to the neurosensory retina.
Melanin, the best absorber of all the pigments, is located in the RPE and the pigmented choroid. The shorter the wavelength, the greater the melanin absorption. It has a maximal absorption of 400 to 700 nm. The greater the fundus pigmentation, the greater the absorption of light and the more intense the burn for a given amount of power. Thus, in treating a lightly pigmented fundus with laser, one may require a higher power setting.
39.3 Delivery Systems
Three main retinal delivery systems are in clinical use today: the slit-lamp biomicroscope with or without a contact lens, the LIO with a condensing lens, and the endophotocoagulation probe inserted through a pars plana incision. Selection of the appropriate delivery system for treatment will depend on the location to be treated, the setting in which the system is to be used (e.g., clinic vs. operating room), patient comfort, and physician preference.
39.3.1 Slit-Lamp Biomicroscope
The slit-lamp biomicroscope is the most commonly employed delivery system today. The essential components of a functional system are a laser source, an attachment system that connects the laser source to the slit lamp, and a slit-lamp microscope to direct the laser beam to the target tissue.
Photocoagulators are differentiated based on their wavelength, active medium, attachment system, pulse duration, power, and spot size selection system. Photocoagulators are available with a variety of wavelengths. The attachment system may be a fiberoptic cable or an articulated mirror arm. The fiberoptic cable offers greater flexibility, whereas the mirror arm offers maintained beam structure with smaller spot sizes. Spot size selectors are of two varieties, parfocal and defocal. In the parfocal system, the spot size is not changed by altering the focal plane of the treatment beam. Instead, changing the magnification varies the spot size. With the defocal system, the spot size is increased by anterior displacement of the focal plane of the treatment beam. Most photocoagulators today have a mixed spot size selector system, with the parfocal system used in the 50 to 200 µm range and the defocal system in the range greater than 200 µm.
The slit-lamp delivery system can be used with or without a contact lens. The majority of posterior segment applications require a contact lens that serves as a coupling device for laser application. A variety of contact lenses are in use today that differ in magnification, spot size, and field of view. These are important parameters in determining the appropriate lens for a particular area of treatment. The conventional Goldmann three-mirror lens has been used for both anterior and posterior segment applications since biomicroscopic laser photocoagulation began. 21 Its limited field of view at any given instant led to the development of newer lenses to optimize treatment of specific posterior segment structures.
Lenses in use are classified as positive (concave) or negative (convex). The various Mainster lenses and the Rodenstock panfunduscopic lens are positive lenses that create a real, inverted image. The Goldmann and Krieger lenses are negative lenses that create a virtual, erect image. Inverted-image lenses provide a considerably greater field of view than erect-image lenses, permitting treatment of large areas of the retina while maintaining visualization of the optic disc and macula to ensure proper orientation.
Mainster et al 22 compared the magnification, spot size, and field of view of four commonly used lenses: Goldmann three-mirror, Krieger, Rodenstock panfunduscopic, and Mainster standard retinal laser lenses. The Goldmann and Mainster standard lenses have similar magnification, whereas the Rodenstock panfunduscopic and Krieger lenses have 24 and 29% less magnification, respectively. The Mainster standard, Krieger, and Rodenstock panfunduscopic lenses have a working field of view greater than that of the Goldmann lens by 58, 8, and 84%, respectively. The working field of view is the maximum field obtained with a 15-degree tilt of the lens to either side of the optical axis. The field of view of each lens varies with the refractive status of the eye (increased in myopic eyes and decreased in hyperopic eyes). The actual size of the retinal burn varies from the spot size setting of the biomicroscope depending on the specific contact lens used. For the Goldmann, Mainster standard, Krieger, and Rodenstock panfunduscopic lenses, the actual retinal spot size is 8, 5, 53, and 41% greater, respectively, than the aerial spot size setting. 22
The Mainster high-magnification laser lens provides 1.25× magnification and is useful for the treatment of macular lesions. It provides a 75-degree field of view with a 43-degree stereoscopic field of view. The retinal spot size is 20% less than the actual spot size setting. The Mainster wide-field lens provides a 125-degree view with 0.68× magnification and is useful for panretinal photocoagulation (PRP). The retinal spot size is 47% greater than the spot size setting.
39.3.2 Laser Indirect Ophthalmoscope
Since its introduction in 1981, the LIO has become an integral part of most vitreoretinal practices. 16 Its wide field of view and versatility offer many advantages over the traditional slit-lamp delivery system. It is available to deliver argon, krypton, and diode irradiation. It expanded the scope of laser photocoagulation by allowing PRP for patients unable to position at a slit lamp. It facilitates the ease of photocoagulation in eyes with intraocular gas or air. It provides an alternative to cryotherapy for the treatment of peripheral retinal breaks. Studies have indicated that photocoagulation with the indirect ophthalmoscope is as effective as cryotherapy for the treatment of retinopathy of prematurity. 23 The energy efficiency of the diode laser allows greater portability, making it the ideal treatment of choice in neonatal intensive care units.
Disadvantages of the LIO include instability of the optical delivery system and a five- to sevenfold decrease in magnification compared with the slit-lamp delivery system, resulting in variations in burn size and intensity. 24 Additionally, Friberg 25 demonstrated that the precision of burn placement to the intended target is accurate only to ±200 µm. When using the LIO with scleral depression, less power is necessary to create a retinal burn. The variability in burn size, intensity, and placement minimizes the utility of this procedure for macular photocoagulation, but it is ideally suited for treating the peripheral retina.
The retinal spot size depends on the distance of the ophthalmic surgeon from the patient, the power of the condensing lens, and the patient’s refractive status. The retinal spot size increases as the power of the condensing lens increases and as the plus power of refraction of the eye increases. Vitreous substitutes also affect spot size; the presence of air and gas results in decreased spot size, whereas silicone and perfluorocarbon cause an increase in spot size. Scleral depression, by decreasing the posterior nodal point of the eye, results in a decreased spot size.
39.3.3 Endophotocoagulation
Endophotocoagulation is a technique whereby energy is delivered to the retina via a fiberoptic cable inserted through a pars plana sclerotomy. Early endolaser systems using xenon required placement of the probe in close proximity to the retina, and they could not be positioned through intraocular gas because this resulted in thermal damage to the probe. 26 Because of these limitations, the xenon system has been largely abandoned.
The introduction of the argon laser for endophotocoagulation was an important event in the evolution of vitreous surgery. 27 Laser, with its inherent lack of beam divergence, has increased the safety of endophotocoagulation by permitting treatment at a safe distance from the retina. In addition, it has allowed treatment through a gas-filled eye.
During the past several years, considerable advances have been made in endoprobe technology, especially with the diode laser, with an increase in range of functions of each individual endoprobe. Endoprobes incorporating laser capability with aspiration and infusion function are now available and eliminate the need for repeated forays into the eye with separate instruments. 28 In addition, endoprobes combining diode laser capability with illuminating fiberoptics permit a clear view of structures that may have been obscured through the operating microscope. 29
Endophotocoagulation has made numerous vital vitreoretinal surgical maneuvers possible, including internal drainage of subretinal fluid, retinectomy, and retinotomy. The intraocular laser delivery system has increased both the scope and safety of vitreous surgery.
39.4 Techniques and Settings
39.4.1 Settings
Three interrelated factors control the amount of energy that arrives at the tissue: laser power, spot size, and pulse duration. The wavelength determines the tissue to which energy is delivered.
Wavelength
For retinal therapy, the laser must be able to penetrate the ocular media (cornea, aqueous, lens, and vitreous) to interact with the target tissue in the posterior segment. There is 75 to 90% transmission of electromagnetic radiation in the visible range (wavelength of 400 to 1,064 nm) through the ocular media. Most lasers for photocoagulation emit radiation in the visible portion of the electromagnetic spectrum, with the exception of the diode and long-pulsed Nd:YAG lasers that emit in the infrared portion. By utilizing a wavelength of light that is selectively absorbed by the target tissue, sufficient heat is generated to selectively coagulate the target tissue. To maximize the desired therapeutic effect while minimizing surrounding tissue effects, one can select a laser with a wavelength of emission that matches the absorption characteristics of the target tissue (Table 39-1).
Laser | Wavelength (nm) |
Argon | |
Blue-green | 488 |
Green | 514 |
Krypton | |
Yellow | 568 |
Red | 647 |
Tunable dye | 570–630 |
Frequency doubled Nd:YAG | 532 |
Diode | 805 (780–850) |
Nd:YAG | 1,064 |
Argon Green
The argon laser was initially a blue-green laser emitting 60 to 70% blue light. The blue-green laser had several disadvantages, principally associated with the blue wavelength: increased scatter and absorption by cataractous lenses, uptake by macular xanthophyll to cause undesired inner retinal damage, and potential for photochemical toxicity. For these reasons, the argon green laser has largely replaced the blue-green laser for the treatment of retinal vascular abnormalities and macular photocoagulation. Green light is well absorbed by melanin and hemoglobin and poorly by xanthophyll, thereby producing a cone-shaped lesion sparing the inner retina. 30 It is good for direct vessel coagulation, resulting in shrinkage around vessel walls, and hemoglobin heating, resulting in formation of thrombi. Argon green is a commonly employed laser for PRP.
Krypton Red
Krypton red is well absorbed by melanin but poorly absorbed by hemoglobin. Additionally, it is minimally absorbed by xanthophyll and, thus, may be useful in the treatment of subretinal neovascularization adjacent to the fovea. Krypton red light has the advantage of better penetration than argon green through hazy media and mild vitreous or shallow subretinal hemorrhages. Because of its longer wavelength, krypton red penetrates more deeply than argon green and, as a result, applications may be more painful.
The main difference between argon green and krypton red laser light in retinal photocoagulation is that argon green light is well absorbed by hemoglobin, whereas krypton red light is minimally absorbed. Thus, argon green light may produce primary damage to CNV, whereas the thermal effects of krypton red light probably result from light absorption and heating in pigmented tissues surrounding the new vessels and not from direct absorption of light by hemoglobin. Adequate melanin is required in the vicinity of the CNV to produce thermal coagulation of the abnormal vessels. Moreover, a hemorrhage resulting from krypton red laser treatment may be difficult to stop because of poor absorption by hemoglobin.
Dye Yellow
Tunable dye lasers have output that can be tuned over a wide range of wavelengths. The advantages of dye yellow laser include minimal scatter, low xanthophyll absorption, and little potential for photochemical damage. Moreover, it is maximally absorbed by oxyhemoglobin, with the highest oxyhemoglobin-to-melanin absorption ratio and a high oxyhemoglobin-to-deoxyhemoglobin absorption ratio. 31 Dye yellow appears useful for destroying vascular structures while minimizing damage to adjacent pigmented tissue, and therefore many surgeons prefer it for the treatment of retinal vascular and subretinal neovascular lesions.
Diode (Infrared)
Diode lasers emit in the near-infrared range, demonstrating clinical similarity to the krypton red laser. The laser energy is able to penetrate through cataracts and has better penetration through vitreous hemorrhage than the argon laser. Additionally, the diode laser demonstrates excellent penetration through serous fluid and retinal edema. The 800-nm diode laser requires more power and longer exposures to achieve photocoagulation. Moreover, there is less absorption in the RPE and choriocapillaris, resulting in penetration of energy to the deeper choroid.
Power
The output of photocoagulation lasers is expressed in units of power (watts), whereas the outputs for photodisruption and ablation lasers are expressed in units of energy (joules). The power (watts) is equal to the energy (joules) divided by the time period (seconds). The shorter the time over which a given amount of power is delivered, the lower the safety window and the greater the risk for tissue rupture and hemorrhage. Although up to 1 W may be necessary for photocoagulation depending on the laser and ocular media, several hundred milliwatts is generally sufficient for most purposes.
Spot Size
Irradiance, the power per unit area measured in watts per square meter, increases as the spot size decreases. Smaller spot sizes are more affected by dissipation of heat to surrounding tissue and, hence, higher irradiance is needed to achieve the same central effect as with larger spots.
A spot size of 50 µm can be used to target individual vessels or in the presence of media opacity, whereas spot sizes of 50 to 100 µm are generally used for photocoagulation of the macula and peripheral retina, respectively. Small spot sizes have decreased safety windows and can result in inadvertent choroidal rupture and hemorrhage.
Contact lenses used for delivery of laser energy to the posterior segment can also affect spot size significantly. It is important to understand the effect on spot size of the variety of commercially available contact lens systems. Selecting the appropriate lens and spot size setting is necessary to achieve the desired effect while minimizing complications. For example, the Goldmann and Mainster standard lenses have spot size settings that correlate closely with the actual size of the retinal burn produced. However, the Rodenstock, Krieger, and Mainster wide-field lenses produce retinal lesions that are larger than the spot size setting by about 40 to 50%. The disparity should be kept in mind and laser settings adjusted when these lenses are used.
Pulse Duration
For a given power, decreasing the pulse duration can alter the tissue reaction. Short pulse durations may result in photodisruption, whereas longer pulse durations may lead to photocoagulation. The amount of light energy is proportional to the duration of the burn. If the duration is increased, the power should be reduced. Photocoagulation is classically performed at pulse durations of 100 to 200 ms, but recent studies have shown that shorter pulse durations of 10 to 100 ms can result in clinically efficacious burns with an increased speed of treatment. Pulse duration can be increased to about 500 ms in cases of ocular media opacity.
39.4.2 Techniques
Before Laser Surgery
Patients should have a complete work-up, including medical history. The ocular examination should include best-corrected visual acuity (BCVA), slit-lamp biomicroscopy, and indirect ophthalmoscopy. Ancillary imaging testing such as fluorescein angiography, color fundus photography, optical coherence tomography (OCT), and indocyanine green angiography can be performed depending on the underlying diagnosis. When all the data have been collected and the diagnosis has been made, the physician should consider possible treatment alternatives, keeping in mind the natural history of the condition. If the condition is amenable to laser, the physician should weigh the potential benefits and risks of laser therapy.
The physician should discuss with the patient and family the patient’s condition and the risks and benefits of laser therapy in comparison with the natural history of the condition. Once the patient has been informed and has elected to undergo laser treatment, the patient should read and sign an informed consent form that describes the treatment objectives, risks, benefits, and alternatives.
Depending on the laser procedure, imaging studies can be projected to help direct treatment. Before laser treatment, the involved eye is typically dilated with 2.5% phenylephrine and 1% tropicamide. In patients with poor pupillary dilation, 10% phenylephrine can be used.
Treatment
The laser is turned on and allowed to warm up. The spot size, wavelength, and pulse duration are selected, and the power is set below the expected appropriate power level. The patient is then taken to the slit lamp, where the eye is anesthetized with a drop of proparacaine or tetracaine ophthalmic. Once the eye is anesthetized, care is taken to adjust the slit lamp so that the patient is comfortable, with the chin on the chin rest and forehead against the bar. Often, a head strap is used to remind patients to keep their head still and up against the bar during the procedure.
Once the patient is in the appropriate position, the intensity of the light beam and aiming beam are adjusted so that they are visualized clearly and excessive glare is avoided. Next, the foot pedal is adjusted so that it is in a comfortable range, and a test fire in the standby position is performed to prepare the patient for the sound made by the laser. A contact lens is then placed on the eye, and the fundus is examined through the contact lens, with the physician noting the landmarks and area of pathology. For macular laser procedures, the fundus is compared with the angiogram and foveal landmarks are localized carefully so that they can be recognized easily during treatment. When the physician is ready to initiate therapy, the control panel is switched to the on position and treatment is begun. Frequent reorientation with fundus landmarks is a good idea to ensure the proper retinal areas are treated.
Peripheral Scatter Laser Treatment Principles
Panretinal photocoagulation (PRP) is performed with the slit-lamp biomicroscope and contact lens or the LIO with a condensing lens. Several retinal vascular conditions may be treated with peripheral scatter laser, including most commonly diabetic retinopathy, retinal vein occlusions, sickle-cell retinopathy, hyperviscosity syndromes, retinal embolization, radiation retinopathy, ocular ischemic syndrome, retinopathy of prematurity, familial exudative vitreoretinopathy (FEVR), retinal vasculitis, uveitis, Eales’ disease, sarcoidosis, chronic retinal detachment, carotid-cavernous fistula, Coats’ disease, retinal artery macroaneurysms, and incontinentia pigmenti. The rationale for PRP is that it reduces the stimulus for neovascularization through destruction of hypoxic retina, especially the metabolically active photoreceptors, and may improve oxygen perfusion to the remaining viable retina. 32
PRP is typically performed with a semiconductor, argon green, or krypton red laser. An initial complete treatment consists of applying 1,000 to 2,000 medium-intensity burns in the peripheral retina for 360 degrees. Burns are spaced one-half to one spot width apart and are distributed from just outside the disc and temporal vascular arcades to the periphery. To minimize complications, laser spots should not be applied directly to major retinal vessels, posterior ciliary arteries and nerves, intraretinal hemorrhages, or large neovascular fronds.
Classically, PRP was given in two or more sessions to reduce patient pain and postlaser exacerbation of macular edema. However, the Diabetic Retinopathy Clinical Research Network (DRCR.net) conducted a nonrandomized, prospective, multicenter clinical trial comparing PRP in one session and four sessions and found no clinical difference. While the central macular thickness (CMT) was slightly higher and vision slightly worse at 3 days and 4 weeks postlaser in the one-session group, the primary end point of 34 weeks showed a reversal, with the four-session group having a CMT 8 µm thicker and visual acuity two letters worse than the one-session group. 33 If PRP treatment is split into multiple sessions, burns should first be placed in the inferior retina to avoid delay in treatment should a vitreous hemorrhage occur before the next treatment session.
Laser parameters for the slit-lamp biomicroscope include a 200- to 500-µm-diameter spot size and a 10- to 200-ms pulse duration. A power setting of about 200 mW is used to start and titrated up in 20- to 50-mW increments until a burn of the desired intensity is achieved (Fig. 39-3). The power used will vary depending on the media clarity, presence of retinal edema, and degree of fundus pigmentation. Laser therapy is typically titrated to a visible clinical effect (graying or whitening of the retina), which corresponds to necrosis of the photoreceptors and, at higher settings, to the inner retina.

When the LIO is used for PRP or any other retinal laser work, care should be taken to line up the laser beam from the indirect source with the condensing lens to the retinal target. A 20-diopter condensing lens should be used to minimize the spot size in hyperopic eyes or eyes with vitreous substitutes, such as silicone oil. A 28-diopter lens can be used to magnify the spot size in eyes with high myopia and in gas-filled eyes.
Macular Laser Treatment Principles
In general, macular laser work is either localized, ablative treatment for discrete lesions, such CNV, or light “focal” or “grid” therapy for intraretinal edema associated with conditions such as diabetic retinopathy or branch vein occlusions. The mechanism by which focal or grid laser photocoagulation reduces macular edema is unclear. Possible explanations include: a laser-induced reduction in retinal blood flow; replacement of compromised RPE cells with new, healthy cells; increase in the inner retinal oxygen supply; and reinforcement of the outer or inner blood–retinal barriers by stimulation of the proliferation of endothelial cells in capillaries and venules overlying the laser spot. 34
There are several requirements for wavelength selection in macular photocoagulation, including: effective transmission through the ocular media; low absorption by macular xanthophyll; and penetration through subretinal fluid, retinal edema, or thin layers of intraretinal and subretinal hemorrhages. The techniques and laser parameters for various conditions are described in the section on indications later in this chapter.
After Laser Surgery
Once treatment is complete, the laser is turned to the standby position and the contact lens and head strap are removed. The eye can be rinsed. The laser parameters, including wavelength, power, pulse duration, spot size, and number of treatment spots, are documented in the patient’s chart. Instructions including postoperative expectations and cautions are discussed, and the patient is scheduled for a postoperative appointment.
39.5 Complications
Laser photocoagulation, as with any surgical procedure, may be associated with complications. Proper patient selection, patient education, and laser parameters can minimize the most serious complications of excessive energy or misdirected light.
Misdirected light can result in burns of the cornea, iris, and lens in addition to inadvertent burns of the fovea. Anterior segment burns can occur during the use of contact lenses with mirrors to treat the peripheral retina. In addition, they can occur when the LIO is used; thus, when the LIO is used and laser burns become more sporadic, photocoagulation should be stopped and the cornea and lens should be inspected for burns. 35 Anterior segment burns with the LIO result from poor focus. Burns of the iris may result in iritis, accommodative difficulties, or posterior synechiae. 36 Focal lens opacifications occur most frequently in eyes with a senescent crystalline lens after the absorption of light by lens pigments. This can be avoided by using longer wavelengths, such as krypton red.
Although highly effective clinically at halting angiogenesis, laser photocoagulation can lead to unwanted side effects, including significant discomfort during application, 37 permanent retinal scarring, laser spot enlargement postoperatively, rupture of Bruch’s membrane and development of CNV, scotoma, exudative retinal detachment, optic neuritis, RPE tears, subretinal fibrosis, visual field loss, dyschromatopsia, nyctalopia, and decreased peripheral vision. 38 , 39 Systematic clinicopathological analysis of laser-induced retinal lesions over time has demonstrated that exposures of 100 ms and longer typically produce retinal lesions that affect not only RPE and photoreceptors, but also the inner nuclear layer, ganglion cell layer, and nerve fiber layer (NFL). Arcuate nerve fiber and visual field defects can result from laser lesions that affect the inner retina. PRP can also exacerbate DME. Permanent retinal scarring has multiple detrimental effects: (1) it distorts the normal retinal architecture and replaces it with gliotic/fibrotic matrix; (2) it disrupts normal retinal connectivity; (3) it is associated with an infiltrative/inflammatory process involving cell loss; (4) lack of photoreceptors and/or other retinal cells in the scarred areas directly reduces the visual field sensitivity; (5) loss of one class of retinal cells may, by trans-synaptic degeneration and by incitement of inflammation, result in loss of other retinal neurons. 40 , 41
Inadvertent foveal burns can occur if the eye moves during photocoagulation and the ophthalmic surgeon is not aware that treatment is occurring within the macula, or if the fovea cannot be identified. When using a contact lens with mirrors, the surgeon should frequently check the location of the macula by looking through the center of the contact lens before treating peripheral retina through the selected mirror. Difficulty in determining the center of the fovea that is not resolved with fluorescein angiography may be a contraindication to macular treatment.
Heavy PRP can result in elevated intraocular pressure, choroidal effusion, cystoid macular edema (CME), nyctalopia, and visual field loss. A transient increase of intraocular pressure can also occur. The majority of pressure rises are of an open-angle variety, the etiology of which is not known. Less commonly, heavy treatment can result in choroidal effusion with angle closure and subsequent elevation of intraocular pressure, which may persist for several days. 42 Dividing treatment into several sessions may reduce the risk of this complication.
Direct treatment of retinal blood vessels or retinal neovascularization may result in hemorrhage. Hemorrhages can occur as an early or late complication of laser photocoagulation. Bleeding into the retina and vitreous can occur during treatment with intense power and small spot size; this may stop spontaneously or with direct laser therapy or digital pressure. Immediate or delayed hemorrhage can occur with photocoagulation of CNV. Immediate bleeding can occur at the area of photocoagulation or from a remote site, whereas delayed bleeding typically occurs from inadequately treated CNV. Intense, confluent treatment of CNV, including a rim of surrounding retina, should decrease the frequency of this complication.
The treatment of CNV can lead to unique complications, including RPE tears and macular holes. Rips of the RPE have been described, particularly with the krypton laser. 43 These occur most frequently in the presence of a pigment epithelial detachments, and Gass 44 hypothesized that when spontaneous tears occur in pigment epithelial detachments, the detachments are associated with occult CNV. Macular holes have also been described following laser photocoagulation of CNV. 45 It is thought that contraction of the photocoagulation scars results in tangential forces to the fovea, resulting in a macular hole.
Delayed complications of photocoagulation include secondary CNV, subretinal fibrosis, and macular pucker. These complications generally occur in areas where small spot size combined with intense burns has resulted in ruptures in Bruch’s membrane. Contraction of an epiretinal membrane with visual distortion can occur after photocoagulation of retinal vascular conditions, especially those associated with inner retinal hemorrhages. This may be a consequence of damage to the inner limiting membrane.
Complications of laser affect not only the patient but also the ophthalmic surgeon. There is now conclusive evidence that subtle but definite alterations in color vision can result from chronic exposure to argon blue light. 46 Therefore, ophthalmic surgeons should minimize their exposure to argon blue light and all laser light by installing appropriate safety filters, blocking blue laser light, or using a different laser wavelength.
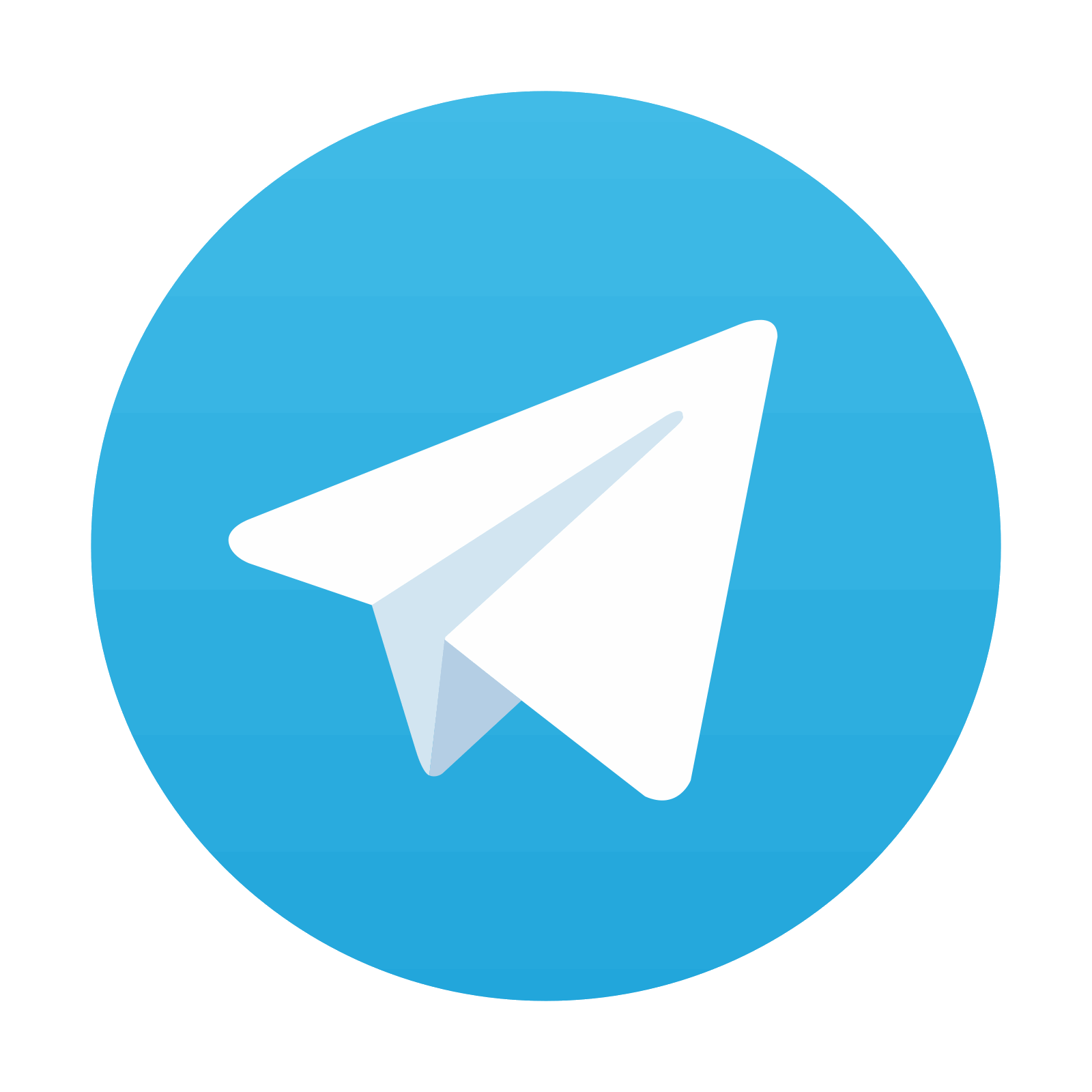
Stay updated, free articles. Join our Telegram channel
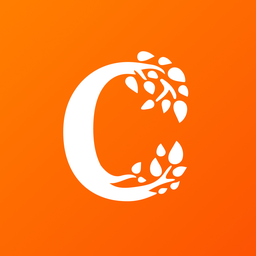
Full access? Get Clinical Tree
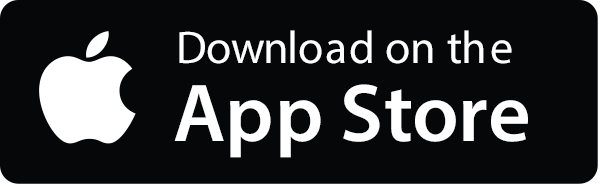
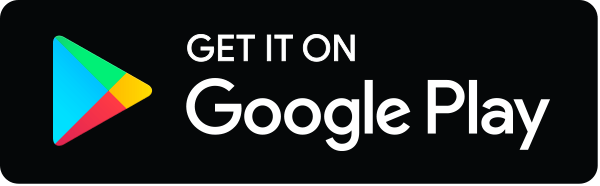