Abstract
Placing an electrode for functional restoration of an injured auditory pathway at the base of the brain requires fundamental understanding of anatomical and physiological constraints. The auditory system has been extensively studied in the past. In fact, it is the single most researched human sensory modality. The following chapter attempts to deliver the massive collection of data on anatomy and physiology of the human auditory brainstem system in a comprehensive for anybody who approaches the topic of a neuroelectronic interface to the process and perceive sounds.
Basic aspects of each anatomical structure in and around the brain-stem auditory pathway, focusing on the cochlear nuclei, but also touching the cochlea, the cochlear nerve, and higher auditory nuclei will be discussed.
The chapter serves as a reference for the biological specifics just as much as an inspiration and guide to overcome obstacles in the development of most effective auditory brain-stem implants.
2 Neuroanatomy and Physiology Relevant to Auditory Brainstem Implants
2.1 Introduction
It stretches human imagination that in a profoundly deaf person speech perception can be partially re-established by a device implanted by a surgeon that activates fewer than 10 effective electrodes placed over a biological structure containing nearly 30,000 neurons. It is even more astonishing if we consider that these neurons are modulated by multiple other neurons, and that the target region cannot be visually distinguished from surrounding neural structures.
To understand why auditory brainstem implants (ABIs) function at all, we will focus on two major aspects here:
Why do present ABI actually work?
How can these neurotechnical interfaces be improved based on what is known about the microanatomy and physiology of the auditory system?
The neural processing of two of the basic physical properties of sound—volume and pitch levels—starts as far peripheral as the cochlea itself. Pitch is transferred from a specific frequency into a spatial location along the hair cells of the basilar membrane and their associated neurons.
The brain deciphers pitch by determining which fibers of the cochlear nerve are maximally active at a given point of time.
Volume or loudness is coded in the cochlea by the firing frequency of these primary sensory neurons. The brain interprets volume as a function of both the number of axons firing and their frequency.
The other function of sound perception, localization, requires bilateral input from both ears, and this function cannot be restored with any unilateral hearing prosthetic.
In this chapter, I will discuss the basic morphological and physiological aspects of each anatomical structure in the brainstem portion of the hearing pathway where the electrodes of current ABIs located as well as the adjacent sites of the hearing pathway (Fig. 2.1). I will delineate opportunities and obstacles for the development of effective ABIs presented by these biological specifics.

2.2 The Cochlea and the Cochlear Nerve
While patients to be implanted with an ABI usually do not have a functional cochlear nerve, it remains important to consider this nerve in order to understand the concept of tonotopy. The cochlear nerve relates cochlear tonotopy to the brainstem. The fibers of the cochlear nerve originate from the nerve cell bodies of the spiral ganglion, in the modiolus of the cochlea. The neurons of the spiral ganglion are the first (of four) order neurons between the cochlea and the cerebrum.
The inner and outer hair cells in the spiral organ (of Corti
Alfonso Giacomo Gaspare Corti, Italian anatomist, 1822–1876.
) are the sensory receptor cells for the mechanical impulses that are primarily generated when sounds hit the eardrum and are transmitted via the middle ear structures to the cochlea. A single inner hair cell is innervated by numerous myelinated nerve fibers, whereas a single—unmyelinated—nerve fiber innervates many outer hair cells. A particular region with a number of hair cells which supply input to a particular afferent nerve fiber is considered to be the “receptive field” of this fiber. High frequencies are represented at the base turn of the cochlea while low frequencies have their receptive field at its apex (Fig. 2.2). This arrangement of neurons in the auditory system is “cochleotopic” or “tonotopic.”
There are different types of hair cells corresponding to two different types of auditory nerve fibers: Inner hair cells convey their signals through type I fibers of the cochlear nerve. Type I fibers are myelinated, constitute 90 to 95% of the fibers in the auditory nerve, and are the primary afferent input to most of the cell types in the cochlear nucleus. 1 , 2
Outer hair cells are innervated by type II fibers, which are unmyelinated and project to nonprincipal cell regions of the cochlear nucleus. 3 , 4 Responses to sound have not been recorded from type II fibers and it is not clear what role they play. 5 Outer hair cells appear to participate in the mechanical response of the basilar membrane; loss of outer hair cells leads to a loss of sensitivity to soft sounds and a decrease in the sharpness of tuning (i.e., the frequency selectivity) of the basilar membrane.
About 95% of the cochlear nerve fibers innervate the inner hair cells. The cochlear nerve trunk exits the modiolus to run into the internal auditory canal (IAC). The peripheral segment of the cochlear nerve joins the vestibular nerve to form the vestibulocochlear nerve (VIII cranial nerve) at the lateral part of the IAC. In cadaver studies, the two portions of the nerve have been found to have a distinct and quite constant spatial relationship: in the IAC the cochlear portion lies anterior to become located inferior to the vestibular nerve in the middle of the nerve and remain there until its entrance into the brainstem. 6 In a magnetic resonance (MR) study, the spatial relationship between the cochlear nerve and the superior portion of the vestibular nerve was more variable. In general, the prevailing—cadaver study based—textbook notion that the three nerves rotate around each other needs to be corrected. It is more accurate to describe the cochlear nerve and inferior vestibular nerve as coursing beneath the superior vestibular nerve in either the IAC or cerebellopontine cistern. 7
Glial tissue surrounds the nerve in the inner ear canal. Close to its exit, the porus acousticus, the glia encasement changes into by Schwann
Theodor Ambrose Hubert Schwann, German anatomist and physiologist, 1810–1882.
cells (“Obersteiner-Redlich zone”). It is here where most vestibular schwannomas originate.The primary terminal of the cochlear nerve is the cochlear nucleus in the medulla oblongata. The length of the vestibulocochlear nerve, from the glial–Schwann junction to the brainstem, is 10 to 13 mm in human males and 7 to 10 mm in females. 8
The pars cochlearis of the human cochlear nerve contains 32,000 to 41,000 fibers with a diameter of 2 to 3 µm, with nearly all myelinated for fast conduction. 9 The fibers reach the ventral cochlear nucleus (VCN) at its ventromedial surface. At the root entry zone, each axon splits into an ascending and a descending fiber. 10 Ascending fibers run dorsolaterally into the VCN, and descending fibers run caudal and dorsal straight through this portion and diverge into the dorsal cochlear nucleus (DCN). In contrast to other mammals, the human DCN is entirely penetrated by these descending fibers, forming a rather homogeneous plexus with the neurons. 10 Therefore, the DCN in humans does not have a laminar structure as in the cat. 11
2.3 Cochlear Nuclei
2.3.1 Two Nuclei in One: The Ventral and Dorsal Cochlear Nucleus
Based on histomorphological criteria, one of the pupils of Cajal, Lorente de No, distinguished a compact ventral and a flatter, longer portion of the cochlear nucleus well before more detailed investigations. 12 On the cellular level, this subclassification has been confirmed. 13 , 14 , 15 , 16 , 17 , 18
The intramedullary portion of the cochlear nerve runs through the VCN resulting in secondary, more macroanatomical descriptive division into a “nucleus cochlearis ventralis superior” (NCVS) and “nucleus cochlearis ventralisinferior” (NCVI). 16 , 19 , 20 , 21
It is important that there are differences in nomenclature between animal and human research because of the spatial orientation of the brainstem: “anterior” in the animal refers to “superior” in the human. The same is true for the pairing of “inferior” and “posterior.”
Another histological subdivision of the VCN in the animal into a “pars anteroventralis” and a “pars posteroventralis” is not recognizable in humans due to the curved course of the cochlear nucleus. 18
2.3.2 Physiology
Animal Research
The cochlear nucleus contains the circuits through which information about sound is coupled to the brain. In the nucleus, fibers of the auditory nerve contact neurons that form multiple, parallel representations of the acoustic environment, each performing a different analysis of the auditory signal. 22 Calculations such as source localization and the identification of a particular sound are separated and performed in parallel as signals travel through the brainstem auditory nuclei.
The modern definitions of the cell types in the cochlear nucleus were developed by Kirsten Osen on the basis of cytoarchitecture in the cat. 23 Incoming auditory nerve fibers bifurcate in the central region of the VCN and send an ascending branch to the anterior division of the VCN and a descending branch to the posterior division of the VCN. The descending branch also curves back to innervate the DCN. To understand the neural ABI interface, it is important to note that the primary terminal of the auditory nerve fibers is the VCN. As we will see below, that is a reason why electrical stimulation with brainstem-penetrating electrodes evoke auditory responses at lower intensities than stimulation with surface electrodes. 24 The innervation of the cochlear nucleus by auditory nerve fibers reflects the tonotopic organization of the cochlea.
The principal cells in the cochlear nucleus are arranged such that each type receives input from auditory nerve fibers over the whole tonotopic range, that is, each principal cell type carries a separate but complete representation of the sound coming to the ear on that side of the head. In projecting to different targets in the brainstem, the principal cell types form separate, parallel pathways. 5 Innervation by auditory nerve fibers both in the VCN and in the deep layer of the DCN is tonotopically organized. In the cat, low-frequency encoding fibers innervate bands ventrally and high-frequency encoding fibers innervate bands dorsally.
McCreery et al proved that it is possible to access the tonotopic gradient of the cochlear nucleus of the cat by electrical stimulation by measuring evoked potentials in different layers of the inferior colliculus (IC) higher up in the brainstem auditory pathway. 25 It is well known that the IC has a layered tonotopy. These researchers demonstrated the tonotopic order in the cochlear nucleus (CN) by stimulating at different sites along its ventro-dorsal axis. Stimulation at various depth in the CN resulted in electrical activity in specific frequency layers in the IC. 25 This finding was an important motivation to design penetrating electrodes for ABIs.
There are at least five primary cell types in the cochlear nucleus, and each has its own unique pattern of response to sound, consistent with the idea that each type is involved in a different aspect of the analysis of the information in the auditory nerve. The diversity of these patterns can be accounted for by three features that vary among the principal cell types: (1) the pattern of the innervation of the cell by auditory nerve fibers; (2) the electrical properties of the cells that shape synaptic inputs; and (3) the interneuronal circuitry associated with the cell. 5
Auditory nerve fibers make synapses on most of the cell types in the cochlear nucleus. Their terminals range in size from small boutons to large endbulbs. 26 Release of neurotransmitters like glutamate activates miniature synaptic currents whose time constants of decay are shorter than 1 millisecond. These kinetics are fast for activation, deactivation, and desensitization; in fact, they match the most rapid that have been measured in any neural structures. Conductances of single glutamate receptors on VCN cells average 28 picoseconds. On average 40 to 50 receptors are activated in a miniature synaptic event. 27 , 28 , 29 In responding to sounds, mammalian auditory nerve fibers fire up to 300 action potentials/second in vivo. 30 Synaptic transmission in the cochlear nucleus is remarkable in showing little plasticity, a feature that is useful for transmitting ongoing acoustic information faithfully and with minimal distortion by preceding sounds. 5
In addition to the frequency information, natural auditory stimuli also contain information on its temporal structure, which is especially important in speech perception. In experiments in which the information encoded in the frequency content of sound is removed, leaving only the temporal structure, much speech discrimination can still be made. 31 This is important since as with cochlear implants, ABIs convey much information via the temporal structure of stimulation. 32 Cochlear nucleus neurons are sensitive to temporal fluctuations and—compared to auditory nerve fibers—generally sharpen the representation of temporal information, to changes in stimulus amplitude. 33 , 34
With different response patterns, these neurons act to separate specific pieces of information from sound stimuli. For example, “onset neurons” show the largest enhancement of the temporal sound structure. The representation of temporal and spectral information encoded in various types of neurons allows the identity of sounds to be determined, that is, one speech sound versus another.
The important aspect with respect to auditory implants is that aspects of the acoustic environment are separated out at the brainstem level, and that they are selectively processed and represented in the cochlear nuclei.
There are even more sophisticated mechanisms of feature detection in the mammalian DCN. Principal cells of the DCN integrate inputs from auditory nerve fibers and from parallel fibers that carry a mixture of auditory and nonauditory information. In contrast to the VCN, where effects of inhibition are relatively weak, DCN neurons in unanesthetized animals receive strong inhibition from both sets of inputs. Spectrally complex sounds evoke a summation of excitation and inhibition that enables neurons to detect spectral features, which are often the information-bearing elements of sounds. 35 , 36 DCN principal cells seem to signal “interesting” features in the stimulus spectrum, by being inhibited where such features lie near the best frequency. 5
The second set of inputs to DCN principal cells conveys multimodal sensory information, from the somatosensory spinal nuclei. A number of researchers have suggested that the DCN is involved in coordinating motor and sensory information in sound localization and that it might be performing a role similar to one kind of cerebellar learning. 5
All parallel ascending auditory pathways through the brainstem converge in the IC. Some fibers of the cochlear nerve send signals from the brainstem (olivary nucleus) back to the cochlea (efferent feedback) which reduce the sensitivity of the cochlea in the presence of loud sounds, reducing saturation. 37
2.4 Human Research
Functional data about the auditory brainstem nuclei in humans are scarce. In the 1970s Dublin attempted to establish a “cochlear nucleogram” to chart tonotopy in the cochlear nuclei. He measured the loss of spheric cells in the VCN and of spiral ganglion hair cells in postmortem specimens and correlated these with the estimated audiogram in sensorineural hearing loss to determine a “best fit” frequency map (“audiohistogram”). His data revealed an intranuclear gradient with low frequencies represented ventral and high frequencies dorsal. 19 , 38 , 39 These and other findings basically matched the tonotopic map that was found in animal research. 11 , 18 , 40
The next step in charting tonotopy in humans came with the advent of ABIs when pitch ranking became possible by selectively activating stimulating electrodes along the brainstem surface of the cochlear nucleus. Pitch ranking showed the lower frequencies to be represented more medial (deeper inside the so-called “lateral recess” of the fourth ventricle) and caudal, and higher frequencies more lateral and cranial. 41
The significance of these data, however, is arguable since the inner (medial) electrodes primarily stimulate the DCN and electrodes located on the outer (lateral) portion of the carriers overlap and may stimulate primarily the VCN. Moreover, the location of the electrodes varies inter-individual and, as we will see, most of the VCN cannot be reached by surface implants at all. In addition, one must bear in mind that the distribution of the electric field around the electrodes is inconsistent, so that it is not easy to calculate at what depth neurons underlying specific electrodes would be excited or inhibited by the electric stimulation. Again, it is notable that threshold-distance measurements were similar in human and animal research. 42 , 43 When it comes to charting tonotopy, however, the possibility that perceived pitch will also be a function of depth penetration of the electrical field generated by surface electrodes, is detrimental.
The key point demonstrated by these studies is that axons conveying higher frequencies penetrate to terminals deep inside the VCN while axons representing lower frequencies terminate more superficial. 10 , 18 , 19 , 44 In the human DCN, fibers carrying higher frequencies terminate more ventral than fibers carrying lower frequencies. 10 , 18
Another line of functional data on the human cochlear nucleus stems from degeneration studies. Axons in the cochlear nerve have a trophic function for the second-order neuron of the auditory pathway that originates in the cochlear nucleus. It has been shown that following destruction of the cochlea resulting in deafness, neurons in the DCN degenerate much less than those in the VCN. 11 , 45 This suggests that most of these neurons do not primarily receive their input from the cochlear nerve.
In a group of deaf patients suffering from sensorineural hearing loss, Jean Moore and colleagues found a 20 to 30% shrinkage of cells in the VCN in postmortem studies. They found the same effect in a deceased patient with a cochlear implant, suggesting that electrical stimulation alone cannot substitute for the trophic function of afferent nerve fibers. 46
Interestingly, the authors saw the same cell shrinkage in higher centers of the auditory pathway, namely the olive and the IC. The necessity of trophic input from the cochlear nerve, however, is limited, since 70% of the cochlear nucleus cells do not show signs of degeneration even a long as 10 years after the onset of deafness. Again, data from human and mammalian animal research overlap considerably. 47 Still, data from both sources are of limited use for the surgeon tasked to place an implant as close to the auditory brainstem nuclei as possible.
2.5 Anatomy and Surgical Approach
2.5.1 Extrinsic Anatomy of the Cochlear Nuclei
In contrast to the favorite auditory research animal, the cat, only a small portion of the ventral human cochlear nucleus lies superficial. 42 The cochlear nucleus itself is not visible on the surface of the human brainstem. However, there are some structural patterns that are helpful in guiding surgeons during ABI implantation. Neurosurgeons have become more acquainted with the surface anatomy of this particular brainstem area, with attempts made to correlate intrinsic anatomy to outer landmarks such as the “torus” and the “auditory tubercle.”
“Tuberculum auditivum.”
, 48 , 49 , 50In the 1980s, researchers of the House Ear Institute (HEI) in Los Angeles reported that part of the wall of the lateral recess of the fourth ventricle is formed by the cochlear nuclei, and that the dorsal surface of the DCN—with the exception of a tiny caudal portion—is almost entirely accessible from the surface of this region of the brainstem. 20 , 21 , 51 Jacob et al measured this accessible area inside the lateral recess to be 7.5 × 2.5 mm. 52
Anatomically, a 0.5-mm-thin cell layer of the so-called pontobulbar body, a relay station between the brainstem and the cerebellum, can overlap the dorsal aspect of the DCN. 42 More recently, Abe et al revisited this anatomical region and showed that the DCN, which lies back to back with the cerebellar peduncle, forms a slight elevation on the surface of the brainstem—the “auditory tubercle.” 53 This elevation, however, is probably only partially formed by the DCN; the pontobulbar body, the vestibular nucleus, and the ependymal lining of the fourth ventricle may be responsible for another portion. 55 , 56 Therefore, and because it is not even present in each case, 49 the auditory tubercle is not a reliable surgical landmark, even with normal anatomy.
The “visible area of the cochlear nucleus” on the surface of the brainstem has been reported to be 11.7 ± 2.7 × 3.1 ± 0.7 mm. 23 Moreover, the mean distance between the auditory tubercle and another visible structure on the surface in this area of the brainstem, the median sulcus, has been measured to be 6.5 to 6.9 mm, 49 while the mean distance between the DCN and the median sulcus is about 10 mm. As such the auditory tubercle is longer than and lies medial to the DCN. 54
“Nuclear torus” is a term that is used for a more extended eminence on the surface of the medulla oblongata that is supposed to be created by the DCN, the lower part of the VCN, and the root entry zone of the vestibulocochlear nerve. This makes it a structure that curves around the posterior lateral border of the brainstem. 52 Both the auditory tubercle and the torus have been measured by these researchers. The mean length of the torus was 12.8 mm. The length range of the tubercle was reported to be 3.5 to 9 mm and its height (rostrocaudal extension) 1.2 to 3 mm.
The most striking result of these definitions and measurements is their vagueness and variability. This holds true for the cochlear nucleus itself, too. Abe et al note that the VCN may reach the entrance of the lateral recess of the fourth ventricle (foramen of Luschka). However, since most of the VCN is located deep inside the brainstem, it is unlikely that this structure causes much of an outer landmark on the surface. Moreover, it is difficult to define a clear border between DCN and VCN even in histological section.
More interesting may be the distance from the apparent origin of the borders of the VCN to the facial nerve, because this nerve usually is, even in cases of neurofibromatosis, often well visible during surgery in this area. While the DCN is located below the facial nerve exit zone in most cases, the more deeply located VCN stretches right across the apparent origin of this nerve from the brainstem. This would make access to the VCN from the outer surface of the brainstem problematic. Notably, the cochlear nerve enters the middle portion of the VCN at its root entry zone (Fig. 2.4). 56


The classical landmark for the surgical introduction of a surface electrode into the lateral recess is the foramen of Luschka. Komune et al have precisely described the anatomy around this entrance into the lateral recess (Fig. 2.3). They used the term “rhomboid lip” for the ventral border of the foramen, a structure that is probably consistent with the lateral part of the taenia of the choroid plexus. 48 , 49 , 57 At the HEI, Terr and Edgerton created three-dimensional models of the cochlear nucleus based on light microscopy analysis in cadaveric specimens to determine the optimal surgical approach to the cochlear nucleus complex (CNC). 20 , 58 They showed that the terminal part of the cochlear nerve approximately coincides with the line of attachment of the taenia of the choroid plexus (= inferior medullary velum of the fourth ventricle).
The glossopharyngeal nerve guides the approach to the foramen of Luschka and it also serves as an estimate of the trajectory for the implantation of a surface ABI electrode array. Klose and Sollmann noted that in two-thirds of cases, a branch of the lateral pontine vein runs over the entrance of the lateral recess (Sollmann’s vein, Fig. 2.3). 48 In formalin-fixed specimens, the foramen of Luschka has a size of about 3.5 × 2 mm. 48 , 49
2.6 Intrinsic Anatomy of the Cochlear Nuclei
It has been suggested that the volume of the cochlear nucleus almost triples over the first five decades of life, and then decreases again by about one-third until toward the end of life. 59 Seldon and Clark found a larger volume of the human cochlear nucleus on the right side. 45 This may be attributed to a preference of the right ear in right-handed people. 60 , 61 , 62 The fact that neurons in the hearing cortex of the dominant left hemisphere have been found to be larger than on the right side would be well in line with this finding since a majority of fibers of the auditory pathway cross over to the contralateral side. 63
The inferior cerebellar peduncle, a large neural fiber connection between the pons and the cerebellum, borders on and partially overlaps the most ventral portion of the cochlear nucleus (Fig. 2.4). 10 , 11 , 18 The floccular peduncle, which originates from the cerebellar flocculus, forms part of the lateral border of the VCN. From the caudolateral portion to its ventrolateral aspect, the VCN is crossed by myelinated fibers (“peripheral astrocytic border”) of the pontobulbar body. This nucleus is a neuronal relay site with connections to visual and auditory regions of the cerebellum, the trigeminal system, and to the spinal cord. 18 , 64 , 65
For several reasons, the real dimensions of the human cochlear nucleus are difficult to assess. In histological sections, the borders of the VCN and DCN are not clearly delineated. Also, histological fixation ultimately leads to shrinkage of specimen in the range of 10 to 17%, more pronounced in longitudinal than in transversal direction, so that measurements need to be multiplied by a correction factor. 46 , 54 , 59 , 66 Until recently, the intrinsic morphological dimensions of the cochlear nucleus in the human brainstem and measurements of protruding structures have only been examined in case studies. 18 , 21 , 49 , 53 , 67 On magnetic resonance imaging (MRI) the nucleus is hardly detectable, but its length and width on a horizontal plane have been estimated to be 8 × 3 mm. 68
Previous attempts to determine the dimensions of the cochlear nucleus have resulted in varying results. Measured rostrocaudal dimensions have ranged from 2.3 to 4.5 mm. 13 , 17 , 45 , 52 , 69 Moore and Osen were the first to investigate the shape and the spatial orientation of the human CNC. 18 They noted that its rostrocaudal (“up to down”) axis is tilted to the brainstem axis by an angle of 30 to 35 degrees. Other authors attempting to better refine this have come up with sometimes paradoxical results. 70 , 71 , 72 Other attempts were made to measure the distance of the nucleus from the surface of the brain over its length. 20 Needless to say, measurement of this complex structure is far from a simple issue.
In order to attempt to synthesize the complex issue of dimension, spatial orientation, and depth of the cochlear nucleus, we undertook a three-dimensional study of 20 brainstem specimens (33 nuclei). 54 The most striking finding in this analysis was that the inward rotation of the VCN to the longitudinal axis of the brainstem is so pronounced that the rostral half of the VCN has a distance of several millimeters to the surface of the brainstem (Fig. 2.5).

The overall extension of the CNC as a whole was 8.01 × 1.53 × 3.76 mm (length × width × height) with respect to the intrinsic axis of the complex, with standard deviations between 0.2 and 1.21 mm (Table 2.1). The VCN becomes wider in more rostral (upper) slices of the brainstem and its longitudinal axis (length) was longer in more caudal (lower) sections.
Length | Width | Height | |
DCN | 3.42 ± 1.21 | 0.68 ± 0.20 | 1.90 ± 0.66 |
VCN | 4.59 ± 0.89 | 1.53 ± 0.64 | 3.18 ± 0.69 |
CNC | 8.02 ± 1.05 | 1.53 ± 0.64 | 3.76 ± 0.89 |
Abbreviations: CNC, cochlear nucleus complex; DCN, dorsal cochlear nucleus; VCN, ventral cochlear nucleus. |
Individual variability of the dimensions of the cochlear nucleus is high. In this study, the smallest and largest values for maximum dimensions and maximum surface depths varied by factor of 3 (Table 2.2). Of note, there was no single CNC that was smallest or largest in all three dimensions.
Length | Width | Height | ||||
Smallest | Largest | Smallest | Largest | Smallest | Largest | |
DCN | 1.46 ± 1.00 | 4.24 ± 1.67 | 0.29 ± 0.19 | 0.81 ± 0.19 | 0.77 | 3.10 |
VCN | 1.92 ± 1.11 | 5.42 ± 1.75 | 0.780 ±.31 | 2.10 ± 0.91 | 2.32 | 4.26 |
CNC | 3.38 ± 1.05 | 9.66 ± 3.42 | 0.78 ± 0.31 | 2.10 ± 0.91 | 2.32 | 7.36 |
Abbreviations: CNC, cochlear nucleus complex; DCN, dorsal cochlear nucleus; VCN, ventral cochlear nucleus. |
In each CNC, width and length of the VCN and DCN correlated with each other, that is, if the DCN was smaller in these dimensions, the VCN was too. Interestingly, the height of the DCN correlated inversely with the length of the VCN and that of the whole CNC.
In this particular study, there were no significant side differences with respect to length and height of the cochlear nucleus. On the contrary, most data regarding these variables and the surface depth of the nuclei on either side of the brainstem correlated linearly with each other. The only exception was the mean length of the DCN in the sagittal plane: it was greater on the left side than on the right. With advancing age, the height of the CNC was reduced, a finding that correlates to the pattern of degenerative shrinkage without reduction in the number of cells described in the literature. 47 , 56
There are few data on three-dimensional shape of the human cochlear nuclei. At the HEI, Terr and Sinha have produced two models, one demonstrating the CN in relation to the brainstem and the other showing the CN completely dissected out of the brainstem (Fig. 2.6). 65 Of course, measures of variance are unobtainable with this method, but still the models helped to design the first ABIs. In our molded three-dimensional renderings of the CNC, it has a distorted X-like or bootlike shape viewed from the side and looks like a wedge from above (Fig. 2.7). 54 , 55 , 73


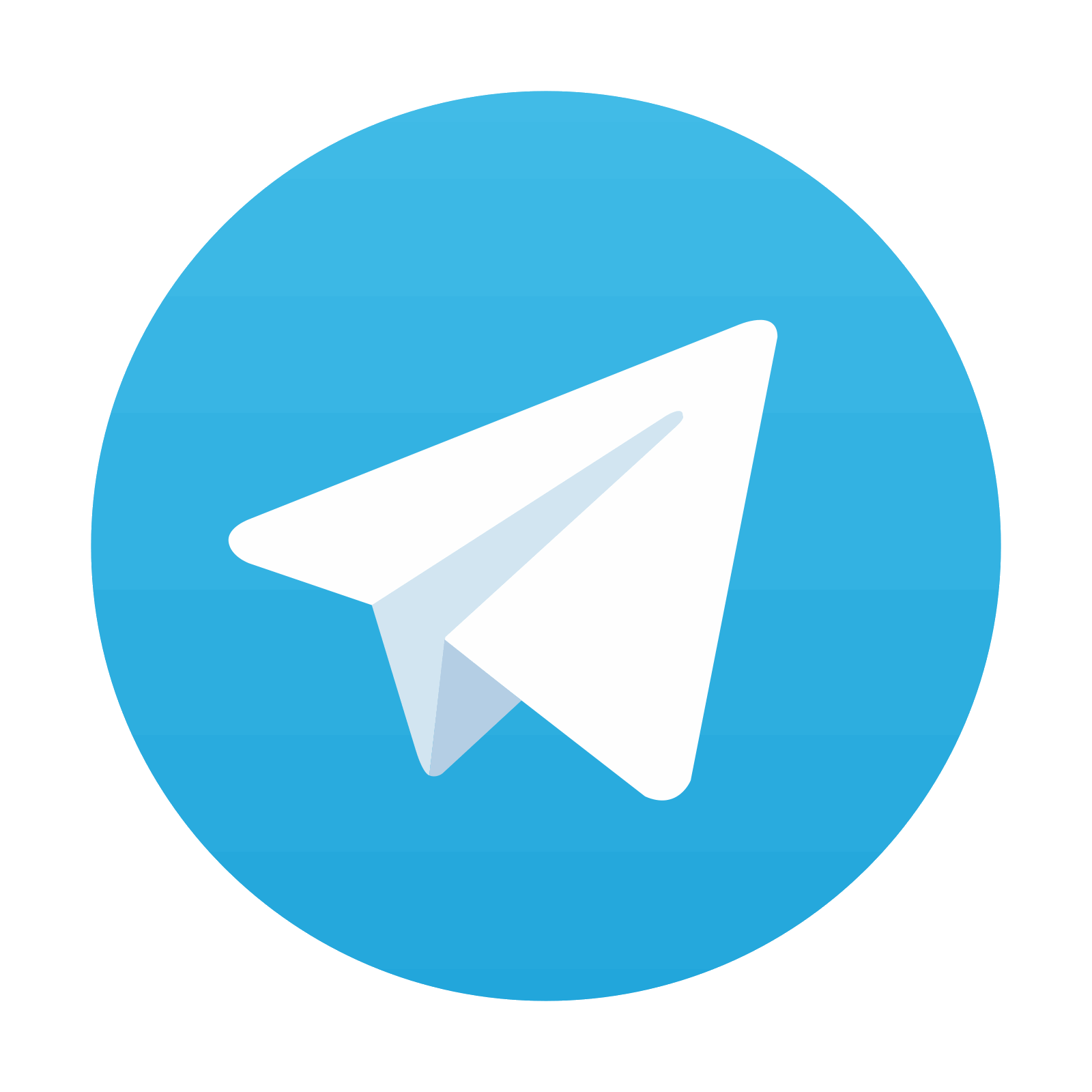
Stay updated, free articles. Join our Telegram channel
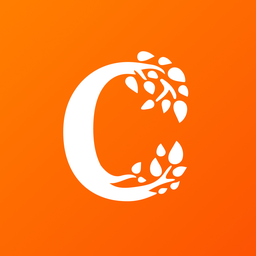
Full access? Get Clinical Tree
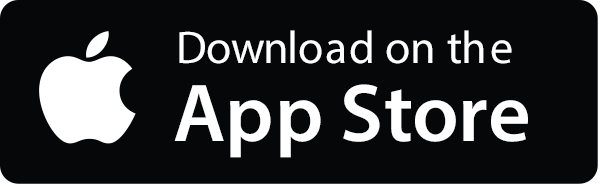
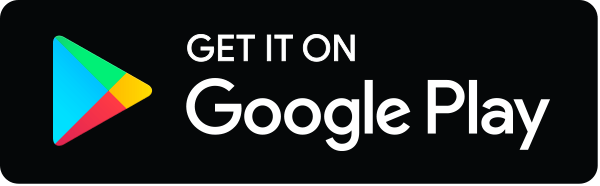