The limited behavioral repertoire of the infant and the impossibility of instructing the test participant have made it necessary for vision scientists interested in human visual development to adapt the classical methods of psychophysics and electrophysiology for use with infants and preverbal children. These methodological adaptations and their interpretation are considered in the context of a hierarchical model of visual processing that organizes the discussion of empirical studies of development at different levels of processing.
Methodologies for assessing infant vision and their interpretation
Preferential looking
Infants’ spontaneous visual fixation is attracted to certain stimuli more readily than to others. In particular, infants prefer to look at patterned stimuli rather than regions of uniform brightness. This spontaneous behavior has served as the basis for a quantitative measure of stimulus visibility known as forced-choice preferential looking (FPL). In the FPL task, the infant is confronted with a randomized series of patterns of varying visibility, presented either on the left or the right of a test screen. An observer judges whether the infant’s fixation behavior is biased to the left or the right on a trial-by-trial basis. If the observer’s judgments agree (or disagree) systematically with the actual position of the stimulus, it can be said that the infant’s behavior is under the control of the stimulus. Distributions of the observer’s judgments for a series of stimulus values are used to plot a psychometric function that relates the observer’s percent correct to the stimulus values presented to the infant. Thresholds are estimated by curve fitting and interpolation to a criterion value of percent correct.
Visual evoked potentials
Visual evoked potentials (VEPs) are electrical brain responses that are triggered by the presentation of a visual stimulus. VEPs are distinguished from the spontaneous electroencephalogram (EEG) due to their consistent time of occurrence after the presentation of the stimulus (time-locking). For example, the abrupt contrast reversal of a checkerboard pattern consistently produces a positive potential on the scalp at a latency of approximately 100 ms in adults. Time-locked responses to abrupt presentations are referred to as transient VEPs . A second method of recording VEPs, the steady-state method, uses temporally periodic stimuli. For commonly used pattern reversal stimuli, the frequency of the repetition is often specified as the pattern reversal rate in reversals per second. This rate is twice the stimulus fundamental frequency (in Hz), which is more commonly used to describe the temporal frequency of pattern onset-offset stimuli. As the stimulus repetition rate increases, the responses to successive stimuli begin to overlap. At high stimulation rates, the response comprises only a small number of components that occur at exact integer multiples of the stimulus frequency. Activity at each of the frequency components of the steady-state response is characterized by its amplitude and phase, where phase represents the temporal delay between the stimulus and the evoked response.
The surface-recorded VEP reflects the activity of cortical visual areas, with contributions from subcortical generators being apparent only under highly specialized recording conditions. The primary adaptations of adult VEP recording techniques for infants involve the control of fixation with fixation toys or superimposed video images and the rejection of trials when the infant’s fixation was not centered on the stimulus.
Ocular following movements
Both infants and adults make reflexive eye movements following the presentation of a moving target. Optokinetic nystagmus (OKN) is characterized by a repetitive saw-tooth waveform. Rapid displacement of large fields also elicits short-latency ocular following movements. Ocular following can also take the form of slower, pursuit-like movements. Reflexive eye movements are controlled by a combination of cortical and subcortical mechanisms. Infrared tracking, electro-oculography, and naked-eye observation of the preponderant direction of eye motion (DEM) are the primary assays of ocular following used in infants and preverbal children.
Hierarchy of visual processing
Fig. 38.1 presents the schematic framework of visual processing that will be used to focus the discussion of empirical studies of visual function in infants and young children. The visual processing hierarchy is divided into three stages: early, middle, and late. The progression from early to late correlates roughly with an ascent from the retina to the cortex and with a functional hierarchy corresponding to the complexity of the information extracted at each level. In this view, early vision begins in the retina and continues through the lateral geniculate and on into primary visual cortex. By the level of primary visual cortex, stimulus attributes such as orientation, direction of motion, and disparity have been extracted from the retinal images. Middle vision—the processes by which local measurements of image features such as line orientation are integrated across space—begins no sooner than primary visual cortex and no doubt extends through a number of first- and second-tier extrastriate visual areas. The content of the representation at the level of middle vision includes information regarding the shape of extended contours, figure/ground relationships, the symmetry of objects, and surface depths, but not the identity of the objects in the scene. The identification of objects (object recognition), which involves not only visual perception but also memory, is conceptualized as occurring in higher-order visual and visual association areas functionally associated with “late vision.”

Each of the different methods for assessing visual function in the preverbal child has a different relationship to the visual processing hierarchy. The FPL technique depends on the integrity of the early visual system, as well as additional mechanisms responsible for the spontaneous preference for pattern (labeled “preference generator” in Fig. 38.1 ).Whether or not middle or late mechanisms are invoked may depend on the discrimination the infant is called on to perform. Orienting behaviors could be driven from many levels of the cortical hierarchy or from subcortical structures. In any case, the output of the preference generator must produce robust fixation behavior that can be detected reliably by the FPL observer. Information regarding the location of the stimulus can be lost at the level of early vision, or at the level of the preference generator or by the observer of the infant’s behavior. Given the additional sites for potential information loss after early vision, FPL is a conservative estimator of the function of the early part of the visual pathway.
Like FPL, the VEP depends on the integrity of the retina and an unknown amount of cortical processing. Fixation, in the sense that the stimulus must fall on central retina, is required, but spontaneous orientation to a preferred stimulus is not. Electrical activity in the visual pathway is obscured by non-stimulus-related electrical activity associated with the EEG and muscle activity, as well as electrode-motion artifacts. The obscuring experimental noise can be reduced effectively, either through time-locked averaging or spectral analysis. At this point, relatively little is known about the contribution of extrastriate cortical areas to the VEP. Given this, the VEP is quite likely to reflect the capabilities of early vision, but caution must be used in inferring the integrity of later stages of processing—especially if simple stimuli are used.
Ocular following movements require the integrity of the retina, certainly, but given the substantial role of subcortical mechanisms in the control of eye movements, it is difficult to specifically relate eye movement data to the hierarchy of cortical mechanisms shown in Fig. 38.1 .
This review emphasizes developmental studies that have used the VEP. The rationale for this choice is several-fold. First, there is now sufficient evidence to indicate that the infant VEP is generated after the site of orientation selectivity, direction selectivity, Vernier offset detection, and binocular correlation detection. All these features are considered to be the outputs of early vision. In adults, it has been found that the VEP reflects both rivalry and suppression, as well as several aspects of middle vision, including figure-ground segmentation based on either texture or motion. Second, the VEP does not require visual preference or transfer of information through the observation of spontaneous behavior and is thus less likely to underestimate the capabilities of early vision. Third, the VEP provides a rich source of information regarding the temporal dynamics of the visual response and, through the use of high-density recording arrays, source localization. Finally, there are already excellent reviews that have emphasized FPL and OKN measures of developing visual function. In deciding which studies to include, emphasis has been placed on those results that have been replicated by more than one research group, wherever possible. Data from the other methods are selectively discussed when these data can help to fill in gaps or when they illustrate particularly sharp contrasts.
Spatiotemporal vision
The retinal images contain a precise spatiotemporal mapping of the visual scene onto two-dimensional surfaces. At the most basic level of processing, the visual system must extract the contrast of the retinal images as a function of time and spatial scale. Visual sensitivity is limited by both spatial and temporal factors. Infant developmental studies have tended to focus on sensitivity along one dimension at a time—by measuring contrast sensitivity as a function of spatial frequency for a fixed temporal frequency or vice versa. Sensitivity depends strongly on both parameters. Whereas the FPL technique can be used at any combination of spatiotemporal frequency, the eye movement and VEP measures each require temporally modulated stimuli. Given the fundamental importance of contrast sensitivity for subsequent visual processing, contrast sensitivity and the related function, grating acuity, are among the few visual functions to have been studied extensively with each of the major methods discussed previously.
Fig. 38.2 plots peak contrast sensitivity as a function of age as determined by the steady-state VEP, DEM, and FPL methods. Each of these studies obtained peak sensitivity measures at a midrange of temporal frequencies (around 5–10 Hz). There is considerable development of contrast sensitivity in each of the techniques, but the absolute contrast sensitivity is higher with the VEP. By 10–14 weeks of age, infant peak contrast sensitivity over the 0.25 to 1 cpd range is approximately 200, which is within about a factor of 2 to 4 of adult levels when measured on the same apparatus. Skoczenski and Norcia found a factor of difference between 10-week-old infants and adults at 1 cpd. Shannon and coworkers found sensitivities at 1.2 cpd that were a factor of about 6 lower than adults at 2 months, with the difference decreasing to a factor of 2.5 at 3 months. Contrast sensitivity measured with the steady-state reversal VEP develops over progressively longer intervals as spatial frequency increases, as shown in Fig. 38.3 .


In contrast to the VEP, several behavioral measurements of contrast sensitivity in this age range show values that are much lower (worse) than adult levels. Rasengane and colleagues reported that low-spatial-frequency flicker sensitivity of 2-month-olds was a factor of 45 lower than adults, with 3- and 4-month-olds being a little less than 20 times less sensitive with FPL. Brown and colleagues used a directional eye movement measure (0.31 cpd grating drifting at 15.5 deg/sec) and found that 3-month-olds were a factor of 100 less sensitive than adults on the same measure. Dobkins and Teller measured both FPL and DEM thresholds in 3-month-olds. They found that infants were almost 30 times less sensitive on the directional eye movement measure and about 60 times less sensitive when FPL and adults’ forced-choice psychophysical thresholds were compared. FPL and DEM thresholds were within 20% of each other in the infants. In adults, DEM thresholds were higher than psychophysical thresholds by a factor of 2 to 3, depending on whether the subject’s task was detection of the direction of motion or simple contrast detection. Peterzell and coworkers measured contrast sensitivity for static gratings in 4-, 6-, and 8-month-olds using FPL and found that sensitivity increased from approximately 8 at 4 months of age to approximately 40 in 8-month-olds, again much lower than VEP sensitivity.
Hainline and Abramov used DEM recorded by an infrared eye tracker to measure contrast sensitivity. The observer made a forced-choice judgment on the output of the tracker (noise level 0.5 degrees) rather than on naked-eye observation. Contrast sensitivity with this method develops to adult levels by 5 months of age (see Fig. 38.2 ).Absolute thresholds are lower than those measured with the VEP by a factor of about 4. Hainline and Abramov’s contrast sensitivities are higher than those observed by Dobkins and Teller or Brown and colleagues who used naked-eye observation at substantially higher luminance. The difference in sensitivities obtained with naked eye and instrumented observation of eye movements suggests that at least some of the lower sensitivity seen in previous behavioral studies may have been due to information loss in the observer who is judging the infant’s behavioral output.
Grating acuity
At the limit of the high spatial frequency limb of the contrast sensitivity function lies the observer’s grating acuity. Grating acuity is limited by the optical quality of the eye, the spacing of the photoreceptors and the spatial pooling properties of the ganglion cells and subsequent receptive field mechanisms. Grating acuity is also limited by temporal factors, being maximal at low temporal frequencies.
VEP grating acuity has been commonly measured using steady-state, pattern reversal targets in the frequency range of 5 to 10 Hz (10–20 contrast reversals per second). The acuity measurement is extrapolated from the high spatial frequency portion of the amplitude versus spatial frequency function. In this method, originally developed by Regan, the spatial frequency of a temporally modulated pattern is systematically changed (swept) over a large range of spatial frequencies that span the expected acuity limit of the observer. Fig. 38.4 plots grating acuity as a function of age for such pattern reversal stimuli. Each study employed the swept spatial frequency technique. Acuity growth functions are similar across studies, with acuity increasing from 4 to 6 cpd in 1-month-olds to about 15 to 20 cpd around 8 months of age, (e.g., within a factor of ~2 of the adult).

VEP acuity has also been measured with pattern onset-offset stimuli, in both transient and steady-state paradigms. Two studies of the transient on-off acuity growth function found that acuity improved from approximately 2 cpd at 1 month to 30 cpd by 5 months. A third study that used checks rather than gratings found an acuity of 2.3 cpd (corrected for Fourier fundamental spatial frequency of the checks) at 8 weeks, with an increase to 8 cpd at 24 weeks. When both transient onset-offset and 6 Hz contrast reversal stimuli were used to measure acuity in the same infants, two different rates of growth were found—transient onset-offset acuity increased 0.63 octaves per month versus 0.28 octaves per month with 6 Hz pattern reversal. The observations that the rate at which acuity increases depends on the response component being measured suggests that different postreceptoral visual mechanisms have different rates of development.
Vernier acuity
Vernier acuity refers to a collection of spatial localization tasks requiring the detection of a misalignment relative to a reference. Adult Vernier thresholds are significantly better than would be predicted based on either the optical or anatomical properties of the eye. Therefore, Vernier acuity is considered one of the hyperacuities. Because cortical processing is believed to be a critical factor limiting the hyperacuities, the time course for the development of Vernier acuity has been investigated with great interest.
Most investigations of Vernier acuity during the first year of life have been cross-sectional, employing behavioral responses to moving stimuli. However, two studies used stationary stimuli. The results of several of these behavioral studies, plotted over the first 6 months of life, are summarized in Fig. 38.5 . On average, Vernier acuity improves by about a factor of 6 to 8 over the first 6 months of life, with the best thresholds recorded to be around 200 seconds, 1.3 to 1.8 log units poorer than adults.

Zanker and colleagues reported that after infancy, performance on their Vernier acuity task became comparable to that of adults by 5 years of age (see Fig. 38.6 ). However, others report that Vernier development is incomplete at age 5 years. Based on the their data from preschool children, Carkeet and coworkers, using a fitting procedure, calculated that Vernier acuity is two times lower than adult levels at 5.6 years of age (confidence interval 3.5 to 6.5 years, see data in Fig. 38.6 ). In a large study of secondary school students, psychophysical grating acuity was found not to change between 11 and 17 years of age, but Vernier acuity improved by a factor of 2. Thus, there is some agreement in the literature that the development of Vernier acuity is incomplete during the early school years and may not fully mature until 18 to 20 years of age.

As noted previously, the majority of infant behavioral paradigms used to investigate the development of Vernier acuity contained motion. Shoczenski and Aslin have demonstrated that temporally modulated stimuli improve the Vernier thresholds of 3-month-old infants and suggest that Vernier thresholds obtained with moving stimuli may be governed by a local motion mechanism rather than a position-sensitive mechanism. Therefore, our understanding of the developmental time course of Vernier acuity and its relationship to the development of other visual functions is potentially confounded by some of the stimuli that have been used in behavioral paradigms to gain the infant’s attention and interest in the task.
The VEP offers a unique solution to this problem. Norcia and colleagues have suggested that by analyzing the separate Fourier components in the steady-state VEP, Vernier response components may be isolated from those arising from stimulus motion. The VEP response to the introduction of a Vernier offset (alignment/misalignment) differs from the response to the return to alignment (misalignment/alignment). This asymmetric response to the introduction and then removal of a Vernier offset is reflected in the odd harmonics of the response to the stimulus modulation. The even harmonics in the evoked potential are produced in response to the symmetrical spatial aspects of the stimulus modulation (e.g., local motion of the offset grating) and may be used to examine motion acuity. The results obtained with a sweep VEP technique are shown in Fig. 38.6 . There is a rapid improvement in Vernier acuity over the first 4 months of life. By 6 months of age, Vernier acuity has improved by about a factor of 7, reaching a threshold of nearly 70 seconds, approximately 1 log unit poorer than the normal adult values obtained psychophysically. Between 6 months of age and 7.5 years, improvement occurs at a slower rate, reaching about 40 seconds, a 1.75 times improvement over the course of about 7 years. After the age of 7.5 years there is another, more rapid improvement in Vernier acuity. Although quantitatively better thresholds were obtained psychophysically by Carkeet and colleagues with stationary stimuli, there is a similar period during the early school years where no significant change in sensitivity was found, followed by a later increase at around age 10 years.
The developmental time course for Vernier acuity has been reported in several studies to be more prolonged than for grating acuity when measured within the same participants. In each of these studies, Vernier acuity is worse in absolute terms in infancy and crosses over to become better than grating acuity, a trend also observed in a study restricted to the infant age range. Vernier acuity also has a longer developmental sequence than grating acuity in macaque. The more protracted development sequence for Vernier acuity is likely due to its critical limiting factors lying in cortex rather than in retinal sampling.
Abnormalities of visual evoked potential (VEP) grating acuity have been found in amblyopia, cortical visual impairment, Down syndrome, spastic cerebral palsy, very low birthweight, and albinism. Exposure to environmental toxins during development (organic solvents and jaundice ) and nutritional status during infancy also affect VEP grating acuity.
Vernier acuity measured psychophysically is often more affected than grating acuity and is better correlated with letter acuity than is grating acuity, a finding that is also seen with the VEP. Consistent with this, VEP Vernier acuity is more affected than is grating acuity in children with moderate to severe cortical visual impairment (CVI). The motion component of the Vernier VEP is preferentially effected in children with CVI who have good visual acuity.
Motion
The detection of motion involves the determination of speed and direction. The presence of direction selective mechanisms early in development has been demonstrated using each of the three major methods, FPL, VEP, and OKN. Directionally appropriate eye movements can be seen at term or even before. Uncertainty remains as to whether these early ocular following responses are controlled by cortical or subcortical pathways. Direction selectivity has been demonstrated behaviorally using FPL and looking time–habituation methods by 6 to 8 weeks. VEP responses associated with changes of direction have been recorded by 10 weeks of age.
Motion direction asymmetries
On any measure, the adult visual system shows roughly equal sensitivity for all directions of motion. Developing infants, on the other hand, show large, systematic biases in their monocular oculomotor and VEP responses. Monocular OKN (MOKN) is robust for nasalward motion but is weak for temporalward motion during the first 3 to 6 months of life as shown in Fig. 38.7 . The time to attaining a symmetric MOKN response may depend on the stimulus velocity, with time to maturity being later for higher image velocities.

Young infants also show monocular VEPs response asymmetries suggestive of a nasalward/temporalward bias in cortical responses. A comparable asymmetry is also present in infant macaques. These response asymmetries manifest themselves in the monocular steady-state VEP made in response to rapidly oscillating gratings. In adults, oscillating gratings produce evoked responses primarily at twice the stimulus frequency (at the rate that stimulus direction changes, F2). In young infants, an additional response component is present at the stimulus frequency (first harmonic response, F1; see Fig. 38.8 ). The first harmonic is 180 degrees out of phase in the two eyes. This pattern of response—a significant first harmonic that is of opposite phase in the two eyes—is consistent with a response bias that is in opposite directions in the two eyes. The absolute direction of the bias, nasalward or temporalward, cannot be directly determined from the steady-state response. It is not known at present whether the cortical motion asymmetry tapped by the VEP causes the oculomotor asymmetry or whether the two phenomena represent immaturities in independent mechanisms with similar developmental sequences.

Symmetric cortical motion responses develop during the first 6 months of life ( Fig. 38.9 ) for 6-Hz oscillatory motion of a low-spatial-frequency grating. Fig. 38.9 plots the ratio of amplitudes at the first harmonic to the sum of amplitudes at the first and second harmonics. This index runs from 1.0 for a completely asymmetric response to 0 for a completely symmetric response. Infants reach adult levels by about 5 months of age for low-spatial-frequency targets oscillating at 6 Hz.

The VEP motion asymmetry has been recorded in infants as young as 2 months of age, suggesting that cortical direction selectivity is present at this time. Interestingly, the motion asymmetry was undetectable in infants younger than 8 weeks at 6 Hz, 1 cpd. Development of motion-specific VEPs over the neonatal period has been observed in a different stimulation paradigm in which the age of first direction-specific responses was also earlier for lower stimulus velocities.
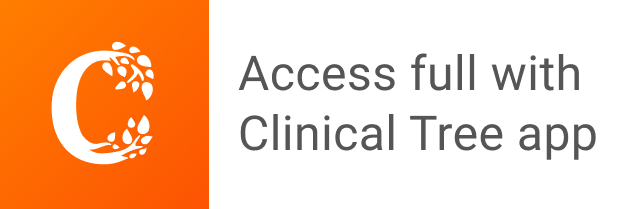